KEY POINTS
-
Soft tissue injuries to the musculoskeletal system are the most common injuries in American football, accounting for ~70% of all visits to physical therapists.
-
One of the reasons for this high rate of injury is that strength, power and speed are dependent on stiff connective tissues, which results in higher rates of injury to the attached musculature.
-
The stiffness of a connective tissue, like a tendon or ligament, is dependent on the collagen content, and the amount of crosslinks within the collagen.
-
Crosslinks increase when athletes train at a high speed or with rapid changes in direction.
-
Crosslinks can be decreased using slow movements that produce greater shear between adjacent collagen molecules, which breaks crosslinks.
-
Connective tissue cells adapt quickly to exercise, beginning to turn off after 5-10 min of activity and requiring a full 6 h to become responsive again.
-
Eating gelatin and vitamin C may promote greater collagen production, especially following a tendon/ligament rupture.
-
By understanding: a) the time course of connective tissue activation, b) the rest period required, and c) the role of nutrition in improving collagen production, a simple training and nutritional plan can be developed to maximize connective tissue function and decrease soft tissue injury.
INTRODUCTION TO SOFT TISSUE INJURY
Musculoskeletal soft tissue injuries (strains and sprains) are the most common injuries suffered by athletes. In American football, nearly 70% of all injuries are sprains or strains (Feeley et al., 2008). This is not simply an issue of the intermittent play or the extreme contact involved in American football, since greater than 60% of all injuries in the English Premier League fall into this category as well (Hawkins et al., 2001). While musculoskeletal injuries are extremely common in elite sports, there have been very few attempts to understand the problem, and as a result, advancements in the prevention and treatment of these injuries have been slow. However, with the huge competitive and financial cost to teams when games are missed due to injury, this issue is starting to garner more attention.
One of the reasons why musculoskeletal injuries are so high in elite sport is that in order to optimize performance, athletes have to maximize the stiffness of the musculoskeletal system. In endurance athletes, stiffness is directly related to their movement economy (Jones, 2002). The same thing is true for power athletes, such as football players since sprinting speed is directly related to leg stiffness (Chelly & Denis, 2001). Therefore, the greater the musculoskeletal stiffness, the better the performance. However, as passive stiffness rises, so does exercise-induced muscle injury (McHugh et al., 1999). Essentially, when the tendon is stiffer than the muscle is strong, the protective effect of the tendon is lost and the muscle ruptures.
The first goal of this Sports Science Exchange is to help develop an understanding of how the musculoskeletal system works and why injuries occur when there is a breakdown in this system. The second goal is to present some data on the types of activity and nutrition that can be used to prevent soft tissue injuries or help athletes return to play faster.
BASIC ANATOMY AND MECHANICS OF THE MUSCULOSKELETAL SOFT TISSUES
When they are working properly, muscles and tendons form a single unit that transfers the force produced in the muscle to the target bone with minimal injury. In order for this system to function properly, it requires the integration of the contractile proteins of the muscle, the intramuscular force transfer apparatus, the intramuscular connective tissue, and the tendon. Each of these separate systems plays an essential role in the development of force and power as well as the health of the muscle.
Muscle produces force when the contractile proteins (myosin and actin) cycle in an energy dependent manner (Huxley, 1967). Even though myosin and actin are the major players, other proteins are needed to hold the structure of the muscle in place (Figure 1). Proteins such as titin, nebulin α-actinin, desmin, the muscle LIM protein (MLP) and the muscle ankyrin repeat protein (MARP) all serve to make sure that the myosin and actin are positioned properly. They also ensure that all of the produced force is transmitted along the muscle length as well as toward the sides of the fiber. As would be expected, these proteins (especially MARP and MLP) increase with resistance exercise to allow better force transfer (Barash et al., 2002, 2004; Woolstenhulme et al., 2006).
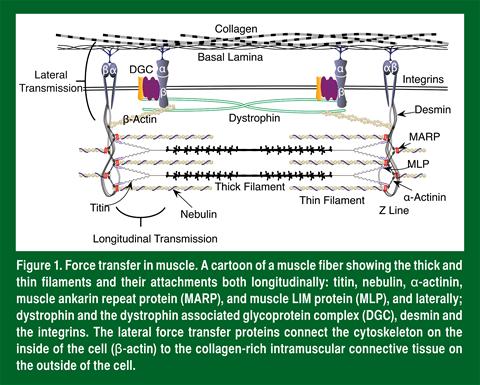
Even though most people believe that muscle develops force by sequential shortening of sarcomeres, in other words transmitting force longitudinally, in reality, greater than 80% of the force developed in a muscle fiber is transmitted laterally to the intracellular connective tissue (Ramaswamy et al., 2011). Lateral transmission has two main functions: 1) to transfer the force out of the working fiber so that it can continue to shorten; and 2) to bind adjacent fibers together to protect them from injury. These two functions make lateral transmission essential to performance since this determines the strength and power of a muscle as well as the likelihood of injury.
Dystrophin is the best characterized of the lateral force transmission proteins and is well known because when it is mutated or absent in young boys, it results in muscular dystrophy, a disease characterized by repeated cycles of injury and repair of skeletal muscle. Without dystrophin, fibers lose one of the key proteins that binds them together and transmits force laterally (Ramaswamy et al., 2011). Therefore, in the absence of dystrophin, fibers function independently and when they slide relative to one another, this opens holes in the membrane that allow calcium to enter and cause degeneration of the fiber (Clafin & Brooks, 2008). Even though dystrophin is essential to force transfer, in young healthy muscle, dystrophin levels do not change with training (Parcell et al., 2009; Woolstenhulme et al., 2006). However, without dystrophin, the other primary force transfer protein complex, α/β integrin, increases in an attempt to compensate (Liu et al., 2012). Like dystrophin, integrins link the actin cytoskeleton on the inside of the fiber to the connective tissue on the outside, allowing the transfer of force and decreasing muscle injury (Boppart et al., 2006). Unlike dystrophin, this mechanical link increases following resistance exercise (Ogasawara et al., 2014), improving force transfer and making it harder to injure individual fibers.
Once the force is transmitted laterally out of the muscle, it is passed to the intramuscular connective tissue. Like other connective tissues, the intramuscular connective tissue is largely composed of fibrillar collagens, elastin and ground substance (Jarvinen et al., 2002). Ground substance is the name given to the sugar-coated proteins that serve to bring and hold water within the tissue. The most abundant protein in connective tissue is collagen and specifically type I collagen. Collagen forms the mechanical backbone of the intramuscular connective tissue and of the tendons and ligaments within our bodies. The strength and stiffness of our connective tissues and tendons is therefore determined in part by the amount of collagen they contain. However, long thin collagen molecules would not be able to transfer force without the chemical crosslinks that bind them together (Marturano et al., 2014). Therefore, crosslinks between collagen molecules are required for a stiff connective tissue that transmits force effectively (Marturano et al., 2014; Reddy et al., 2002). In general, there are two kinds of crosslinks: enzymatic and non-enzymatic. The enzymatic crosslinks are mostly made by lysyl oxidase, a copper-dependent enzyme that links lysine residues of adjacent collagen fibers (Kagan & Li, 2003). Non-enzymatic crosslinks are also called advanced glycation end products (AGEs), since these crosslinks are formed when sugar molecules bind one collagen molecule to another. Since sugar is needed to make these crosslinks, AGEs are much higher in diabetics (Vlassara & Striker, 2013) and higher AGEs results in greater stiffness (Reddy et al., 2002). A practical consequence of this is that diabetics are more prone to musculoskeletal injuries (Ilan et al., 2003).
At each end of the muscle, the intramuscular connective tissue and the last sarcomeres of the muscle come together to form the myotendinous junction (the connection between the muscle and tendon) (Figure 2). This structure is one of the most important for determining the health of the muscle. There are two primary components of the myotendinous junction that protect a muscle from injury: invaginations and orientation. The interface between the muscle and the tendon is not a smooth/flat transition. Instead, finger-like invaginations are present at the interface (Polican Ciena et al., 2012). These invaginations serve two important functions that are responsible for the strength of this tissue. First, the invaginations increase the surface area that connects the muscle and tendon. Second, the invaginations mean that the interface is loaded in shear and not tension. To explain the difference, consider Velcro®. The strength of Velcro® goes up when you have more loops and hooks (increased surface area of attachment) and it is nearly impossible to slide Velcro® fasteners past each other (load in shear). If instead you pull the two pieces directly away from each other (load in tension), the connection is much weaker and they come apart. The same is true at the myotendinous junction. The second important component of the myotendinous junction is its orientation.
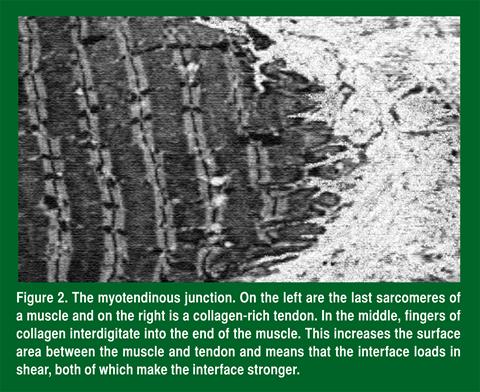
Once the force from the muscle reaches the tendon, a healthy tendon transfers this force to the bone. Since muscle and bone are so different in their mechanical properties (compliant vs. stiff), this simple job (transferring force) is actually quite complex. To transfer the force from a compliant muscle to stiff bone without injury, the tendon has to act as a variable mechanical tissue (Figure 3A). This means that the tendon is compliant near the muscle and slowly becomes stiffer along its length until it nears the bone (Arruda et al., 2006). This is achieved by varying the orientation and crosslinking of the collagen in the tendon. As discussed above, the orientation of the collagen changes rapidly at the myotendinous junction. When the collagen is not as well aligned it is less stiff since it first must align, and second, resist load. Once it aligns, the stiffness increases rapidly. Think of a frayed rope. The individual fibers of the frayed end have only a fraction of the stiffness of the knitted part, largely because they are all oriented in different directions. The second reason that tendons are more stiff near the bone is because in this region they are more crosslinked (Curwin et al., 1994). As discussed above, crosslinking increases stiffness and since there are more crosslinks near the bone than the muscle, stiffness increases as you move from muscle to bone (Arruda et al. 2006). Interestingly, when a joint is inactive (such as when in a cast), the tendons that cross that joint lose the compliant region (Figure 3B) and become stiffer (Arruda et al., 2006), likely because crosslinks increase.
The result of the stiffer tendon is that athletes often suffer injuries to the associated muscle if they return to play too quickly after immobilization.
From the background above, the most important things to take away are: 1) stiffer tendons are better for performance but increase the risk of injury, 2) lateral transmission of force protects individual muscle fibers from injury by linking them to their neighbors, and 3) the compliant region of the tendon acts as a shock absorber and protects the whole muscle from injury. The next important question is, how is the compliant region of the tendon generated?
CELLULAR UNDERPINNING OF GRADED TENDON MECHANICS
As discussed above, tendons have water within them thanks to their ground substance. In fact, over 60% of the mass of the tendon is water and this has important mechanical effects on the tissue. Together, the crosslinked collagen and water mechanically make tendons viscoelastic; they behave as both a liquid and an elastic solid. What this means for an athlete is that the faster a tendon is loaded, the stiffer it acts and the more energy it can store (Figure 4). To understand this concept, think of yourself at a swimming pool. If you are in the shallow end with your hands on the bottom and you lower yourself into the water slowly, you barely feel the transition from air to water. This is because when you move slowly, the water acts more like individual molecules that are easy to move through. If instead you run and jump off the side of the pool and land on your belly, the water acts more like a sheet of connected molecules and as a result seems much stiffer; you feel the entry into the water much more, and come out with a red belly. If you go to the top of the 3-m diving board, jump off and land on your belly the water feels much stiffer still and you are lucky if you don’t end up in the hospital. By extension, when velocity is low, tendons work more like individual collagen fibrils, whereas at high speeds they act as a single unit. This is the likely explanation for how the graded mechanics of the tendon are generated and why this is lost during inactivity.
During slow movements, collagen molecules within the tendon act more independently. At the myotendinous junction (where the collagen invaginates with the muscle) (Figure 2), this means that the collagen near the muscle is pulled differently than its neighbor, resulting in a shear force that likely breaks the crosslinks between the adjacent collagen molecules. As the tendon moves closer to the bone, the collagen becomes better aligned and the shear stress is lost. In this way, active movement breaks crosslinks near the muscle, making the tendon less stiff in this region. If this is true, then immobilizing the muscle should prevent the breaking of crosslinks thus stiffening the muscle end of the tendon, exactly as we see in Figure 3. Therefore, the compliant end of the tendon is produced when shear forces break crosslinks between collagen molecules. This is why slow lengthening contractions are the most effective way to treat tendonopathies (Kingma et al., 2007).
Slowly moving a heavy weight causes more shear and breaks more crosslinks, resulting in the return of the compliant end of the tendon.
In contrast to slow exercises, during fast movements, collagen molecules within the tendon act more as a single unit. As a result, the individual fingers of collagen that invaginate into the muscle are stiffer, stretch less, and this generates less shear stress and breaks fewer crosslinks. In football, where athletes are continuously accelerating, decelerating and colliding, the majority of the sport is composed of these types of ballistic movements that increase the stiffness. This might be why massage and rolling are popular with these athletes since massage can decrease passive stiffness (Huang et al., 2010). However, incorporating slow movements into the training program may have a more beneficial effect in the long run.
Regardless of the type of loading the tendon undergoes (fast or slow), lysyl oxidase (the enzyme that makes new crosslinks) increases more than 20-fold (Heinemeier et al., 2007). The result is that with slow movements you break and re-form crosslinks, keeping the tendon stiffness about the same, whereas with fast movements you add new crosslinks to those that already present in the tissue, making the tendon progressively stiffer. This is why high-speed ballistic movements are best to build power (Foure et al., 2012, 2013).
TRAINING TO IMPROVE SOFT TISSUE FUNCTION
For years, scientists, coaches and athletes considered tendons and ligaments mechanical bands that did not respond to exercise. However, it is now clear that these tissues respond to loading. For example, the patellar tendon in the plant leg of fencers and badminton players is 20-30% larger than their trail leg (Couppe et al., 2008). Since the core collagen in a tendon does not turn over between ages 17 and 70 (Heinemeier et al., 2013), this suggests that a tendon grows like a tree, adding successive rings of collagen that make the structure stronger and more resistant to injury.
Since we can add collagen to our connective tissues, understanding how to exercise to increase the synthesis of collagen and improve the stiffness of the connective tissue is essential to performance. The cells within tendons, ligaments and intramuscular connective tissue do not respond to exercise in the same way muscle does. Unlike muscle that continues to adapt as long as it is active (Baar, 2009), connective tissue cells are more like those in bone and rapidly shut off following the start of exercise (Paxton et al., 2012). Within 10 min of starting an activity, the cells in connective tissue begin to turn off and are shown to take a full 6 h to become responsive to exercise again (Paxton et al., 2012). The result is that, like bone (Burr et al., 2002), tendons make more collagen and become stronger when they are exercised intermittently; 10 min on, 6 h off, 10 min on, and so on (Paxton et al., 2012). A second interesting thing about connective tissue cells is that neither the load (Heinemeier et al., 2007) nor the frequency (i.e., running vs. walking) of loading matters (Paxton et al., 2012). Therefore, even small range-of-motion exercises with a very light weight are effective at increasing collagen synthesis and connective tissue function. This information can be used to prevent injury and accelerate return to play following injury: repeated short periods of activity that load the connective tissue followed by long periods of rest appear to be optimum for connective tissue.
NUTRITIONAL INTERVENTIONS TO IMPROVE SOFT TISSUE FUNCTION
Compared to muscle, the science of nutritional interventions that can improve soft tissue function is in its infancy. One paper has shown that whey protein can improve tendon function (Farup et al., 2014). Other than that, at this point there are no papers that have studied the effect of diet on soft tissue in humans. However, using a tissue engineered ligament model we have shown that certain amino acids and vitamin C can improve collagen synthesis (Paxton et al., 2010). The amino acids in question, including proline, lysine, hydroxylysine and hydroxyproline, are enriched in gelatin, which is made from the tendons and ligaments of cows. We have now begun feeding gelatin to athletes and have seen extremely positive responses on collagen production and return to play in athletes after injury (Shaw & Baar, unpublished). However, larger scale clinical trials are needed to determine the effect of gelatin on tendon collagen synthesis in people.
Even though it is presently unclear whether specific nutrients can positively affect soft tissue function, the physiology of tendons and ligaments suggests that nutritional interventions designed to improve soft tissue function will need to be performed differently than those designed for muscle. Since tendons and ligaments have limited blood flow, they get their nutrients though bulk fluid flow. Here, think of a sponge that gets wrung out and put into a liquid. As the sponge expands, it draws fluid in. The same is true for soft tissues. As they are loaded, the fluid moves out and when they relax, fluid is drawn in from the environment. As a result, the nutrient has to be present in the environment before exercise for the loading to deliver it to the soft tissue.
SCIENCE-BASED RECOMMENDATIONS FOR TRAINING TO MAXIMIZE SOFT TISSUE HEALTH AND PERFORMANCE
From the background provided above, a series of recommendations can be developed to maximize performance and minimize the risk of soft tissue injury.
-
Because of the extreme plyometric load involved in football, slow movements should be incorporated into the training program during the season to maintain the compliant region of the tendon and protect against muscle injury.
-
To further prevent soft tissue injuries, consider incorporating a connective tissue health session into training. This type of session would involve 5-10 min of activity targeted to a specific area that is prone to injury. For example, wide receivers, defensive backs, running backs and linebackers would do a session to target the hamstrings, quadriceps and gluteals, whereas quarterbacks would target the throwing shoulder. These exercises could be done with a light weight and a limited range of motion if necessary. The connective tissue health session should be performed either 6 h before or after any other training.
-
Preliminary work suggests that 30-60 min before both the connective tissue health session and practice, players should be encouraged to consume >2 g of gelatin in either liquid or gel form.
-
In the offseason, more fast movements can be performed in the gym since the weekly plyometric load on the athlete is much lower at this time of year. However, incorporating some slow movements will improve musculoskeletal health as well as provide a greater stimulus for the muscle to grow.
-
Athletes early in the process of recovery from soft tissue injury should be encouraged to perform three separate short training bouts (5-10 min) spaced 6 h apart throughout the day. Incorporating gelatin prior to at least two of these recovery sessions may augment collagen synthesis in tendon, ligament, cartilage and bone, and accelerate return to play.
REFERENCES
Arruda, E.M., S. Calve, R,G, Dennis, K. Mundy, and K. Baar (2006). Regional variation of tibialis anterior tendon mechanics is lost following denervation. J. Appl. Physiol. 101:1113-1117.
Baar, K. (2009). The signaling underlying FITness. Appl. Physiol. Nutr. Metab. 34:411-419.
Barash, I.A., D. Peters, J. Friden, G.J. Lutz, and R.L. Lieber (2002). Desmin cytoskeletal modifications after a bout of eccentric exercise in the rat. Am. J. Physiol. 283:R958-R963.
Barash, I.A., L. Mathew, A.F. Ryan AF, J. Chen, and R.L. Lieber (2004). Rapid muscle-specific gene expression changes after a single bout of eccentric contractions in the mouse. Am. J. Physiol. 286:C355-C364.
Boppart, M.D., D.J. Burkin, and S.J. Kaufman (2006). Alpha7beta1-integrin regulates mechanotransduction and prevents skeletal muscle injury. Am. J. Physiol. 290:C1660-C1665.
Burr, D.B., A.G. Robling, and C.H. Turner (2002). Effects of biomechanical stress on bones in animals. Bone 30:781-786.
Chelly, S.M., and C. Denis (2001). Leg power and hopping stiffness: relationship with sprint running performance. Med. Sci. Sports Exerc. 33:326-333.
Claflin, D.R., and S.V. Brooks (2008). Direct observation of failing fibers in muscles of dystrophic mice provides mechanistic insight into muscular dystrophy. Am. J. Physiol. 294:C651-C658.
Couppe, C., M. Kongsgaard, P. Aagaard, P. Hansen, J. Bojsen-Moller, M. Kjaer, and S.P. Magnussen (2008). Habitual loading results in tendon hypertrophy and increased stiffness of the human patellar tendon. J. Appl. Physiol. 105:805-810.
Curwin, S.L., R.R. Roy, and A.C. Vailas (1994). Regional and age variations in growing tendon. J. Morphol. 221:309-320.
Farup, J., S.K. Rahbek, M.H. Vendelbo, A. Matzon, J. Hindhede, A. Bejder, S. Ringgard, and K. Vissing (2014). Whey protein hydrolysate augments tendon and muscle hypertrophy independent of resistance exercise contraction mode. Scand. J. Med. Sci. Sports 24: 788-798.
Feeley, B.T., S. Kennelly, R.P. Barnes, M.S. Muller, B.T. Kelly, S.A. Rodeo, and R.F. Warren (2008). Epidemiology of National Football League training camp injuries from 1998 to 2007. Am. J. Sports Med. 36:1597-1603.
Foure, A., A. Nordez, C. Cornu (2012). Effects of plyometric training on passive stiffness of gastrocnemii muscles and Achilles tendon. Eur. J. Appl. Physiol. 112:2849-2857.
Foure, A., A. Nordez, and C. Cornu (2013). Effects of eccentric training on mechanical properties of the plantar flexor muscle-tendon complex. J. Appl. Physiol. 114:523- 537.
Hawkins, R.D., M.A. Hulse, C. Wilkinson, A Hodson, and M. Gibson (2001). The association football medical research programme: an audit of injuries in professional football. Br. J. Sports Med. 35:43-47.
Heinemeier, K.M., J.L. Olesen, F. Haddad, H. Langberg, M. Kjaer, K.M. Baldwin, and P. Schjerling (2007). Expression of collagen and related growth factors in rat tendon and skeletal muscle in response to specific contraction types. J. Physiol. 582:1303- 1316.
Heinemeier, K.M., P. Schjerling, J. Heinemeier, S.P. Magnusson, and M. Kjaer (2013). Lack of tissue renewal in human adult Achilles tendon is revealed by nuclear bomb 14C. Faseb J. 27:2074-2079.
Huang, S.Y., M. Di Santo, K.P. Wadden KP, D.F. Cappa, T. Alkanani, and D.G. Behm (2010). Short-duration massage at the hamstrings musculotendinous junction induces greater range of motion. J. Strength Cond. Res. 24:1917-1924.
Huxley, H.E. (1967). Recent x-ray diffraction and electron microscope studies of striated muscle. J. Gen. Physiol. 50:Suppl:71-83.
Ilan, D.I., N. Tejwani, M. Keschner, and M. Liebman (2003). Quadriceps tendon rupture. J. Am. Acad. Orthop. Surg. 11:192-200.
Jarvinen, T.A., L. Jozsa, P. Kannus P, T.L. Jarvinen, and M. Jarvinen (2002). Organization and distribution of intramuscular connective tissue in normal and immobilized skeletal muscles. An immunohistochemical, polarization and scanning electron microscopic study. J. Muscle Res. Cell. Motil. 23:245-254.
Jones, A.M. (2002). Running economy is negatively related to sit-and-reach test performance in international-standard distance runners. Int. J. Sports Med. 23:40- 43.
Kagan, H.M., and W. Li (2003). Lysyl oxidase: properties, specificity, and biological roles inside and outside of the cell. J. Cell. Biochem. 88:660-672.
Kingma, J.J., R. de Knikker, H.M. Wittink, and T. Takken (2007). Eccentric overload training in patients with chronic Achilles tendinopathy: a systematic review. Br. J. Sports Med. 41:e3.
Liu, J., D.J. Milner, M.D. Boppart, R.S. Ross, and S.J. Kaufman (2012). Beta1D chain increases alpha7beta1 integrin and laminin and protects against sarcolemmal damage in mdx mice. Hum. Mol. Genet. 21:1592-1603.
Marturano, J.E., J.F. Xylas, and G.V. Sridharan, I. Georgakoudi, and C.K. Kuo (2014). Lysyl oxidase-mediated collagen crosslinks may be assessed as markers of functional properties of tendon tissue formation. Acta Biomater. 10:1370-1379.
McHugh, M.P., D.A. Connolly, R.G. Eston, I.J. Kremenic, S. J. Nicholas, and G.W. Gleim. (1999). The role of passive muscle stiffness in symptoms of exercise-induced muscle damage. Am. J. Sports Med. 27:594-599.
Ogasawara, R., K. Nakazato, K. Sato, M.D. Boppart, and S. Fujita (2014). Resistance exercise increases active MMP and beta1-integrin protein expression in skeletal muscle. Physiol. Rep. 2:e12212.
Parcell, A.C., M.T. Woolstenhulme, and R.D. Sawyer (2009). Structural protein alterations to resistance and endurance cycling exercise training. J. Strength Cond. Res. 23:359-365.
Paxton, J.Z., L.M. Grover, and K. Baar (2010). Engineering an in vitro model of a functional ligament from bone to bone. Tissue Eng. Part A. 16:3515-3525.
Paxton, J.Z., P. Hagerty, J.J. Andrick JJ, and K. Baar (2012). Optimizing an intermittent stretch paradigm using ERK1/2 phosphorylation results in increased collagen synthesis in engineered ligaments. Tissue Eng Part A. 18:277-284.
Polican Ciena, A., S.R. Yokomizo De Almeida, C. De Sousa Bolina, R. De Sousa Bolina- Matos, R.E. Grassi Rici, M.C. Pereira Da Silva, M.A. Miglino, and I.S. Wayanabe (2012). Ultrastructural features of the myotendinous junction of the sternomastoid muscle in Wistar rats: from newborn to aging. Microsc. Res. Tech. 75:1292-1296.
Ramaswamy, K.S., M.L. Palmer, J.H. van der Meulen, A. Renoux, T.Y. Kostrominova, D.E. Michele, and J.A. Faulkner (2011). Lateral transmission of force is impaired in skeletal muscles of dystrophic mice and very old rats. J. Physiol. 589:1195-1208.
Reddy, G.K., L. Stehno-Bittel, and C.S. Enwemeka (2002). Glycation-induced matrix stability in the rabbit achilles tendon. Arch. Biochem. Biophys. 399:174-80.
Shaw, G. and K. Baar (unpublished). Case Study: Nutrition and training to promote recovery in a Rugby player following ACL rupture.
Vlassara, H., and G.E. Striker (2013). Advanced glycation endproducts in diabetes and diabetic complications. Endocrinol. Metab. Clin. North Am. 42:697-719.
Woolstenhulme, M.T., R.K. Conlee, M.J. Drummond, A.W. Stites, and A.C. Parcell (2006). Temporal response of desmin and dystrophin proteins to progressive resistance exercise in human skeletal muscle. J Appl. Physiol. 100:1876-1882.