KEY POINTS
- Dehydration (>2% reductions in body mass) can impair exercise performance and recovery.
- Cell volume regulation is influenced by both hydration status and skeletal muscle contraction.
- Heat stress during intense exercise may compromise muscle structure and function.
- The effects of dehydration on exercise-induced muscle damage (EIMD) have gone largely unexamined, but limited evidence suggests dehydration may exacerbate EIMD and prolong recovery.
- Proposed mechanisms for the adverse effects of dehydration on EIMD may include alterations in cell volume and ion flux, cell membrane disruption, impaired excitation contraction coupling, decreased skeletal muscle blood flow, modified red blood cell properties, and/or intensification of maladaptive signaling.
INTRODUCTION
Dehydration during training and competition may not only hinder acute exercise performance, but also delay recovery from a previous exercise bout. Recovery is also influenced by the amount of exercise-induced muscle damage (EIMD) that occurs when the structural and functional (i.e., contractile) properties of skeletal muscle are disrupted. This is particularly relevant to athletes and practitioners because recovery is a critical component of performance, especially when multiple practice sessions or competitive events are performed within a short time period.
The effects of dehydration on acute exercise performance have been studied extensively (for review, see Cheuvront & Kenefick, 2014). However, the effects of dehydration on the magnitude of EIMD and subsequent recovery profile have gone largely unexamined. Limited evidence suggests that dehydration may negatively affect indicators of recovery, such as functional performance measures or subjective perceptions of muscle soreness (Cleary et al., 2005; Ozkan & Ibrahim, 2016; Paik et al., 2009; Seifert et al., 2005). Further, multiple mechanisms have been proposed to explain dehydration’s role in EIMD, but human studies are limited. Understanding variables that can accelerate recovery time and optimize recovery strategies may result in a competitive advantage for athletes. Therefore, the aim of this Sports Science Exchange article is to discuss the role of dehydration on the magnitude and duration of EIMD, from the cellular level to the whole body.
BASIC CONCEPTS: EXERCISE-INDUCED DEHYDRATION AND MUSCLE DAMAGE
Exercise-Induced Dehydration
Definitions. Dehydration has been examined as far back as the 17th century and is generally understood as the process of losing body water. Hypohydration refers to the state of water deficit and rehydration is the process of transitioning from a state of hypohydration to euhydration. In this review, dehydration will refer to loss of intracellular fluid as this is the primary form of sweat-induced dehydration. Because sweat is hypotonic in relation to blood, sweat losses increase plasma osmolality and decrease blood volume, which result in hyperosmotic and hypovolemic stressors (Sawka, 1992).
Even though the gold standard for total body water (TBW) determination is often referenced as isotope (deuterium oxide) dilution, dehydration is commonly measured as a function of body mass lost (Cheuvront & Kenefick, 2014). Water losses that exceed 2% of body mass are typically indicated by elevated plasma osmolality, decreased plasma volume and increased urinary biomarker concentrations (Cheuvront & Kenefick, 2014). While body mass measurements are often acceptable in the field as an indicator of hydration status, measuring fluid shifts across muscle cells can provide a greater appreciation of potential mechanisms associated with muscle damage secondary to dehydration. Currently, measuring fluid shifts across cells is not possible in field settings. Therefore, this review will summarize laboratory findings in an attempt to translate experimental data into useful recommendations regarding the potential impact of dehydration on EIMD and recovery.
Fluid Shifts & Volume Sensing. During exercise or environmental heat stress, sweating is initiated to prevent excessive rises in body core temperature (Tc). Depending on the level of dehydration, about 10% of TBW is lost from the plasma, 40-60% from the interstitial fluid (ISF), and 30-50% from the intracellular fluid (ICF) (Costill et al., 1976). However, the extent of water loss from specific tissue compartments is not well understood and may depend on several factors including acclimation status as well as how dehydration is induced (passively through heat stress, exercise alone, a combination of environment and exercise, or pharmacologically) (Kozlowski & Saltin,1964; Sawka, 1992).
Generally, cell volume is well maintained through fluid shifts controlled by osmotic gradients (Sawka, 1992) and ionic pumps (Senay & Pivarnik, 1985). During exercise, muscle cells are challenged to maintain volume despite large fluctuations in metabolic demand, membrane potential and blood flow (Usher-Smith et al., 2009). With moderate and high intensity exercise, intracellular water shifts out of non-contracting skeletal muscle and other inactive tissues, whereas in contracting skeletal muscle there is a net flux of solute poor fluid moving into the skeletal muscle, which further increases plasma osmolality. Subsequently, the non-contracting skeletal muscle cells experience a loss in cell volume or shrinkage, while contracting cells experience an increase in cell volume or swelling in an attempt to obtain osmotic equilibrium (Lindinger et al., 2011).
Changes in cell volume are primarily sensed by osmoreceptors, specialized neurons that detect osmolality and encode this information as electrical signals to send to the hypothalamus (Bourque, 2008). These volume sensors are coupled to mechanisms that cause shifts of osmotic particles across the membrane (Toft-Bertelsen et al., 2018). During cellular dehydration (cell shrinkage) both cerebral and peripheral osmoreceptors contribute to maintaining fluid balance (Bourque, 2008). The exact mechanisms by which cells detect swelling or shrinkage are unknown, but are hypothesized to be related to TRPV channels (a family of transient receptor potential vanilloid cation channels) (Bourque, 2008). Maintenance of cell volume is essential for cell vitality; however, drastic shifts in cell volume caused by dehydration may adversely affect skeletal muscle function and have the potential to augment EIMD.
Exercise - Induced Muscle Damage
The breakdown and remodeling of skeletal muscle fibers is necessary to elicit training adaptations and increase exercise performance. Mechanical theories of EIMD propose that following unaccustomed exercise or high force repetitive eccentric contractions, myofilaments are stretched, and sarcomeres undergo misalignment or “popping” (Morgan & Allen, 1999). Subsequently, the excitation contraction coupling process is impaired by sarcomere disruption, myofiber damage, alterations in membrane permeability and opening of stretch activated channels (Ebbeling & Clarkson, 1989).
The characteristic structural damage associated with eccentric muscle action may be the result of calcium influx through strain activated channels (Ebbeling & Clarkson, 1989). These channels can be activated by the disruption of intracellular calcium stores within the sarcoplasmic reticulum, the T-tubule system or the sarcolemma, followed by activation of proteolytic and lipolytic pathways, especially through calpain activation (Lieber, 2010). Calpain activation results in selective hydrolysis or disruption of the intermediate filament network, preventing the muscle from developing tension and limiting force production. Further, muscle damage creates a distinct inflammatory response that leads to neutrophil and macrophage invasion, occurring simultaneously with the muscle repair and regeneration process (Tidball, 2005). Fibers subjected to this stress may be eliminated or undergo necrosis and regenerate via satellite cells, although there is strong evidence to suggest that tissues may not actually undergo necrosis but rather synthesis and remodeling (Owens et al., 2019). The consequence of this process is well recognized as the delayed onset of muscle soreness (DOMS), presenting as perception of muscle soreness and reductions in exercise capacity described 24-48 h after the precipitating event. Following this cycle of injury and repair, the muscle is better able to withstand future insult and recovery time is accelerated, known as the repeated bout effect (Nosaka & Clarkson, 1995).
Human experimental models of EIMD and DOMS generally include repeated eccentric muscle contractions such as down-hill or prolonged running, drop jumps, high intensity intervals or resistance exercise with emphasis on the eccentric phase. Although physical activity is a combination of concentric, eccentric and isometric muscle contractions, these models mainly utilize eccentric muscle contractions because EIMD is predominantly demonstrated following eccentric as opposed to concentric muscle contractions (Faulkner et al., 1993; Fridén et al., 1986). Recovery from eccentric exercise can be measured both functionally (force produced; range of movement), biochemically (circulating markers of muscle damage or histology) and subjectively (perception of muscle soreness or pain). EIMD is associated with strength loss, perceptions of soreness, and elevations in circulating muscle enzymes or proteins (e.g., creatine kinase (CK), myoglobin). Although not without limitations, these measures are commonly utilized to indicate severity and time course of muscle injury. A decline in muscle force is one of the most reliable indirect measures of EIMD, whereas ratings of muscle soreness, circulating proteins and histology (although a direct measure) should be used judiciously (Warren et al., 1999). Circulating proteins of muscle damage, such as CK and myoglobin, portray a consistent pattern of elevation over a 2-6 day time course, and their appearance in the blood is attributed to increases in membrane permeability (Lieber, 2010). However, direct loss of force follows a more immediate time course and has a different temporal pattern than commonly measured biomarkers (e.g., CK, lactate dehydrogenase (LDH), glutamic-oxaloacetic transaminase, slow myosin heavy-chain fragments, and myoglobin) (Hyldahl & Hubal, 2014). Although different subject populations and modes of exercise have been utilized to develop EIMD, similar patterns of muscle force loss, soreness, dysfunction and biomarkers are apparent across models (Figure 1).
Because of the associated decrements in performance and increased recovery time, EIMD has traditionally been thought of as negative. However, these short term decrements in performance are a reflection of the inflammatory process occurring in the skeletal muscle and the consequential increases in protein turnover that are necessary for long term adaptations to exercise (Lieber, 2010). The optimal stimulus to maximize performance adaptations and optimize recovery is still unknown, and limited research has been done to understand variables that may modify the severity of EIMD, such as dehydration. Evidence describing the influence of dehydration on muscle damage or recovery from eccentric exercise is sparse and inconclusive (Cleary et al., 2005, 2006; Ozkan & Ibrahim, 2016; Yamamoto et al., 2008). Considering that dehydration amplifies the effects of osmotic stress, hyperthermia and skeletal muscle dysfunction, the concept that dehydration may delay exercise recovery should be examined further.
.jpg?sfvrsn=2)
Figure 1. Characteristic time course and recovery pattern of (A) strength loss (muscle maximal isometric strength loss as a percent of pre-exercise values), (B) soreness (visual analogue scale on 50 mm line), (C) plasma creatine kinase activity (IU/L), and (D) plasma myoglobin activity (ng/ml) following two bouts of exercise separated by four weeks recovery. (Reproduced from Hirose et al., 2004; Hubal et al., 2008; Hyldahl & Hubal, 2014, with permission).
MECHANISMS FOR INCREASED SKELETAL MUSCLE DAMAGE WITH DEHYDRATION
Background: Performance & Recovery
The role of dehydration in exercise performance has been extensively studied under a variety of environmental and physiological conditions. Fluid restriction during exercise hinders acute exercise performance, particularly in endurance exercise trials (Cheuvront & Kenefick, 2014). Proposed mechanisms for detriments in endurance performance are associated with reduction in plasma volume, cardiac filling, and stroke volume (Cheuvront & Kenefick, 2014). These adjustments culminate in reduced blood flow to the contracting muscle which alters muscle metabolism and impedes thermoregulation, especially in hot environments. Theoretically, these same adjustments could intensify EIMD and prolong recovery from a bout of exercise.
Although the precise role of acute dehydration on EIMD is unclear, rehydration plays an important role in recovery from exercise. Rehydration has been demonstrated to improve subsequent exercise performance and is especially important following prolonged exercise durations in hot and humid conditions (McCartney et al., 2017). This suggests that proper hydration during the exercise bout may also play a critical role in recovery. Proper hydration may help negate potential processes that can influence EIMD such as the independent and combined effects of osmotic stress, muscle fiber swelling and hyperthermia.
Osmotic Stress
Osmotic stress can be thought of as physiologic dysfunction that is caused by a sudden change in the solute concentration around a cell. During exercise and heat stress skeletal muscle fibers are exposed to multiple fluid shifts both intracellularly and extracellularly that can result in osmotic stress. At the whole-body level, gross dehydration decreases perfusion pressure to the skeletal muscle, reducing blood flow and placing the muscle at risk for ischemia related damage (González-Alonso et al., 1998).
At the cellular level, active skeletal muscle cells undergo acute transient changes in cell volume (swelling followed by shrinkage) signaling reactive oxygen species (ROS) (Laitano et al., 2012). These successive changes across cells may compromise skeletal muscle function, potentially through the regulation of fluid shifts across cells. This signal is likely to be exaggerated when dehydration is concurrent with repeated eccentric muscle contraction and has the potential to increase muscle damage or prolong recovery. Dehydration can alter the redox balance through the effects of ischemia reperfusion on active skeletal muscle blood flow (Laitano et al., 2012). At higher levels of dehydration (4-5% body mass loss), increased blood viscosity augments ROS production through increases in vascular shear stress and red blood cell rigidity (Ahmadizad et al., 2006; Senay & Pivarnik, 1985). Increased ROS generation in skeletal muscle may injure the sarcolemma, cytoskeleton and DNA, as well as lead to the suboptimal alignment of skeletal muscle contractile units, hindering muscle contraction (Steinbacher & Eckl, 2015) (Figure 2). Importantly, ROS production is necessary for adaptation to stressors such as exercise, hyperthermia and dehydration, emphasizing that ROS production should not be avoided completely (King et al., 2016).
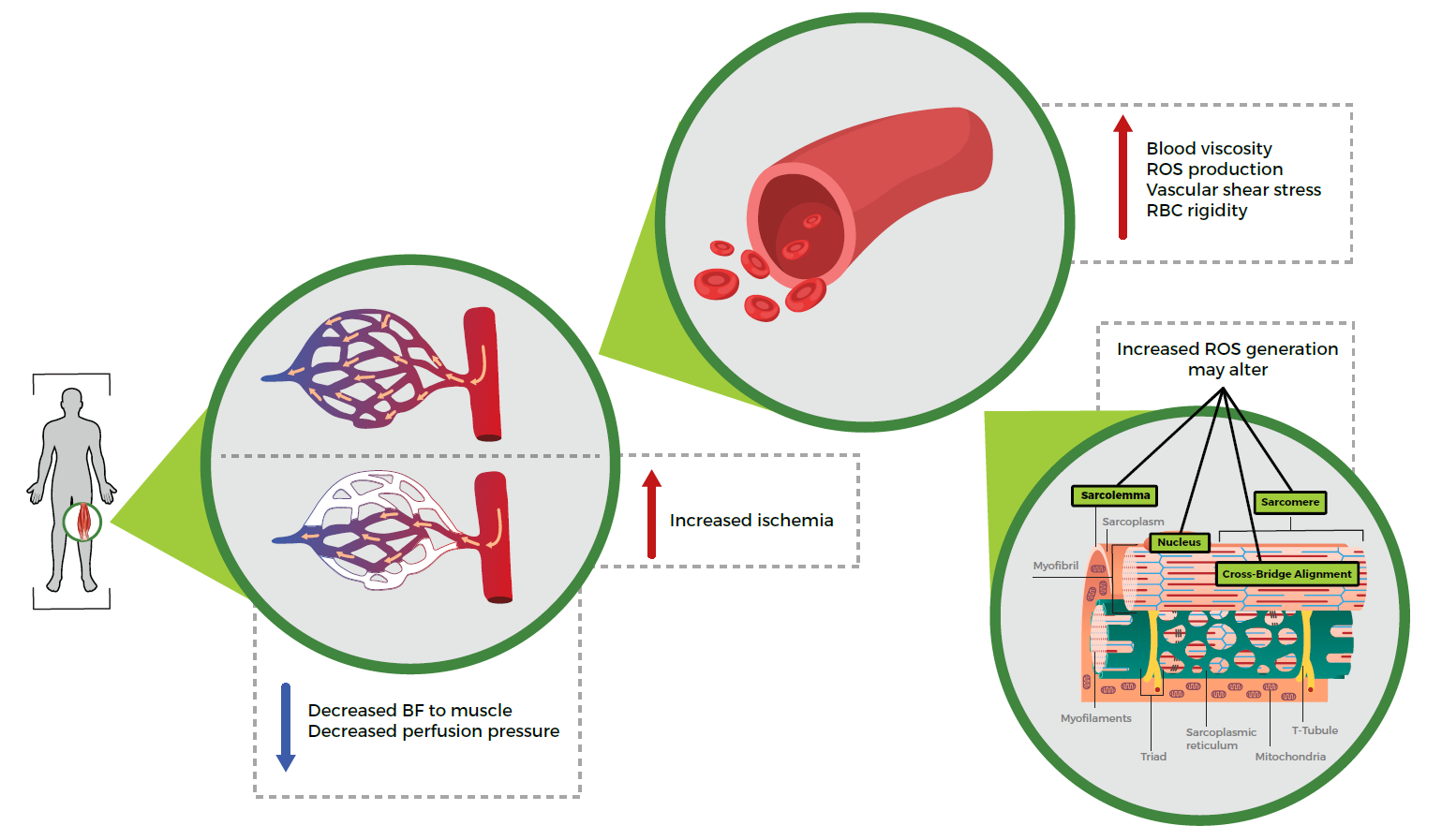
Figure 2. Potential mechanisms for increased skeletal muscle damage with dehydration. BF, blood flow; ROS, reactive oxygen species; RBC, red blood cell.
Muscle Fiber Swelling
Both hyperthermia and exercise can result in evaporative whole-body water loss as well as muscle fiber swelling. During fatiguing exercise contractions, changes in osmotic pressure provide the driving force for muscle swelling, with documented increases in muscle fiber size of up to 35% that may remain for up to an hour following exercise (Usher-Smith et al., 2009). Further, muscle biopsy studies have demonstrated increases in muscle intracellular water content (15%) and cellular volume (15-20%) following maximal exercise (Sjogaard, 1983; Sjogaard et al., 1985). Muscle fiber swelling is caused by elevations in solute content during exercise, where the splitting of phosphocreatine, elevations in lactate and H+, as well as shifts in ion gradients contribute (Hoffmann et al., 2009) (Figure 3). Eccentric muscle contractions appear to cause the greatest amount of fiber swelling, likely a direct result of dysfunctional membrane pumps that lead to decreased sodium extrusion and subsequent swelling of muscle fibers (Fridén et al., 1988). Often overlooked, muscle fiber swelling may be attributed to contraction induced increases in metabolic water production, as water is produced with each gram of carbohydrate (0.6 mL of water) or fat (1.1 mL of water) oxidized (Maughan et al., 2007).
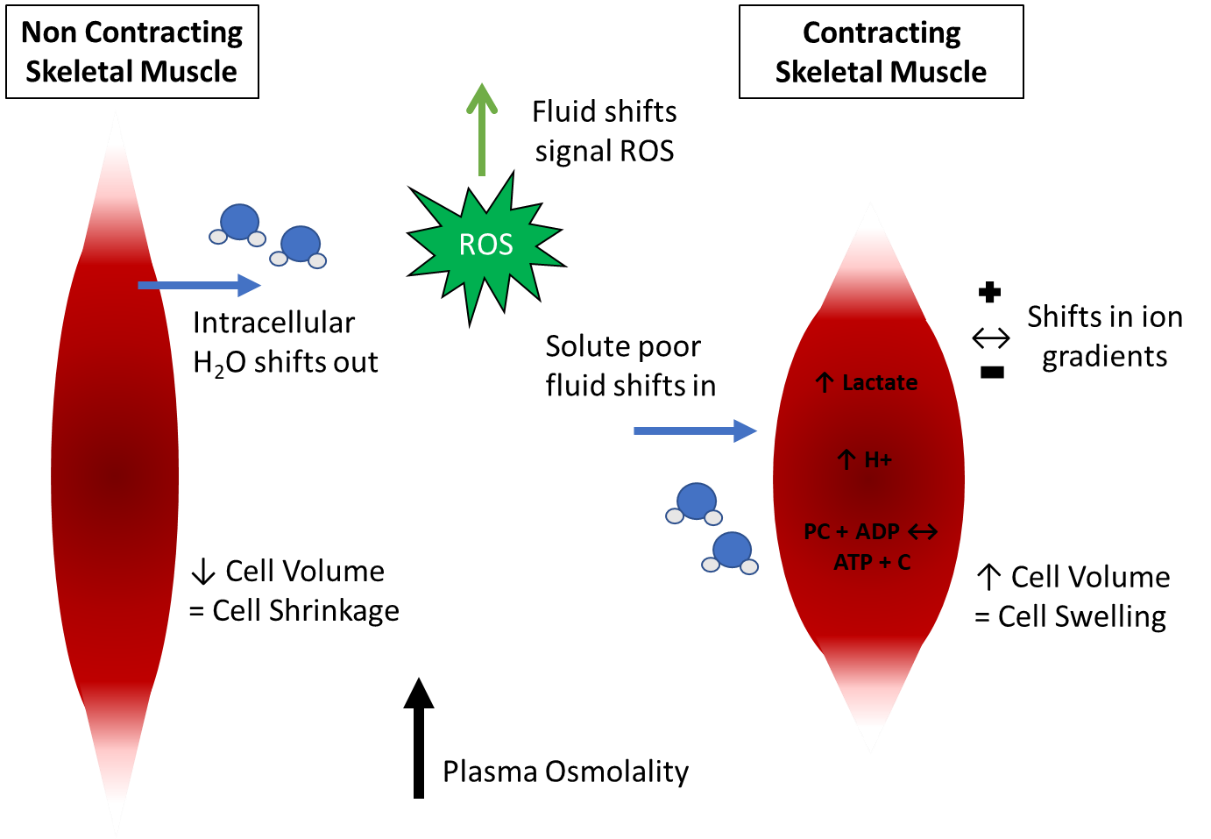
Figure 3. A summary of the consequences of dehydration on fluid shifts during skeletal muscle contraction. ROS, reactive oxygen species.
Additionally, cell culture models demonstrate that hyperthermia independently causes swelling of a variety of cells (Gervais et al., 2003). This cell swelling is associated with reversible increases in the gap between the membranes of the T-tubules and sarcoplasmic reticulum. In the sarcolemma, calcium homeostasis is altered by increases in cation permeability, which allows extracellular calcium influx into skeletal muscle fibers, potentially increasing the immune response and impairing glycogenolysis (Clarkson & Hubal, 2002). Skeletal muscle is particularly susceptible to cell disruption because it relies on intracellular solutes for excitation contraction coupling (i.e., calcium) (Usher-Smith et al., 2009).
Susceptibility to dehydration and EIMD also appears to be muscle fiber dependent. Dehydration may primarily affect fast twitch fibers (Farhat et al., 2018) which are also preferentially damaged by eccentric exercise, (Vijayan et al., 2001) potentially creating an additive effect on muscle damage. However, other research suggests a protective effect of hyperthermic hyperosmolality (simulated dehydration) on sarcolemmal injury, which was also specific to fiber type (Laitano et al., 2018). Because these studies were performed in rodent models, it remains to be determined if these same responses would hold true in humans.
Importantly, a small amount of swelling may enhance skeletal muscle contractile function, whereas severe swelling, such as that seen in osmotic shock, has the potential to detach the transverse tubules from the surface membrane (Usher-Smith et al., 2009). Smaller perturbations in cell volume can also alter muscle cell structure and function by affecting the architecture of the cell, organelles and cell membrane (Usher-Smith et al., 2009). Cell volume is also an important factor in the regulation of protein turnover by signaling protein synthesis during cell swelling and protein degradation during cell shrinkage (Haussinger et al., 1993).
Influence of Hyperthermia
Independent of hydration status, rodent models suggest hyperthermia has a strong influence on the skeletal muscle damage profile and may increase susceptibility to stretch-induced damage (Hill, 1972; Oliver et al., 2008). Muscle temperatures have been shown to reach > 40°C (104°F) during intense exercise (Morris et al., 2005) which can increase passive tension. Increased passive tension places the muscle at a heightened risk for sarcomere damage with greater detriments in strength loss, pain, muscle tenderness, and CK activity (McHugh et al., 1999). Further, hyperthermia itself has been demonstrated to cause a variety of alterations in the skeletal muscle such as cross bridge misalignment, disruptions in muscle lattice structure, calcium release dysfunction, sarcolemma injury and electrolyte redistribution that increases extracellular osmolality and muscle tension (Hargreaves & Febbraio, 1998). A secondary mechanism of injury through proteases and phospholipases may impede calcium mediated calcium release in the sarcoplasmic reticulum further challenging muscular contraction (Owens et al., 2019).
Interestingly, when the independent effect of hyperthermia was isolated via short wave diathermy, heat did not alter functional impairments of the muscle or affect the biomarker profile following eccentric exercise (Castellani et al., 2016). Hillman et al. (2011) isolated the independent effects of environmental heat stress and dehydration on oxidative stress, concluding that exercise-induced dehydration may increase oxidative stress, whereas euhydration is protective of this effect regardless of environmental conditions. There were no independent effects of environmental heat stress besides the expected elevations in heart rate and Tc.
CAN DEHYDRATION INCREASE MUSCLE DAMAGE AND PROLONG RECOVERY?
Understanding elements that are critical to exercise recovery are not only important for athletes and the practitioners that support them, but also for the general population. Although there are plausible mechanisms by which dehydration may augment the extent of EIMD and therefore prolong recovery, current research results are limited and mixed.
When muscle damage was induced via fatiguing resistance exercise (squats), preceded by exercise heat stress and water deprivation, blood biomarker elevations in lactate, CK and myoglobin were similar between dehydrated and euhydrated groups up to 48 h post exercise (Yamamoto et al., 2008). Contrastingly, following multiple bouts of Alpine skiing, CK, myoglobin and cortisol were all significantly elevated when fluid was not replaced during the activity (Seifert et al., 2005). Similar findings were demonstrated when wrestlers utilized a variety of unstandardized dehydration induction methods 1-7 days prior to competition. Dehydrated wrestlers (according to pre-competition plasma osmolarity) displayed higher levels of blood biomarkers (aspartate aminotransferase, blood urea nitrogen, LDH and CK) than the euhydrated groups (Ozkan & Ibrahim, 2016). Although these studies were able to measure blood biomarkers of dehydration and muscle damage, they lacked functional indicators of muscle damage, such as force output or perceptions of muscle soreness.
As discussed above, hyperthermia is known to exacerbate muscle damage, therefore it is important to consider the confounding impact of Tc when interpreting the effects of dehydration on EIMD and DOMS. One key study from Cleary et al. (2005) demonstrated that symptoms of DOMS (pain and tenderness) were exacerbated when dehydration was induced through fluid restriction and environmental heat stress prior to downhill running in thermoneutral conditions. However, a follow-on study found no impact on DOMS when the Tc of the subjects returned to “pre-exercise or normothermic levels” prior to the initiation of downhill running (Cleary et al., 2006). Similarly, Greiwe et al. (1998) demonstrated that when subjects rested following passive heat stress (≈ 4% reduction in body mass) before performing a series of strength measures, dehydration had no influence on serum myoglobin or potassium concentrations, peak torque or time to fatigue. However, the experimental design of these studies did not truly isolate the effects of hyperthermia or dehydration.
CONCLUSIONS AND CONSIDERATIONS
Overall, there are plausible mechanisms by which dehydration during strenuous exercise may augment the extent of EIMD and therefore prolong recovery. However, studies that examined the effect of hydration status on muscle damage or recovery provide mixed results and are limited by experimental design or methodological issues. Investigators have attempted to isolate the independent effects of dehydration from both environmental heat stress and exercise hyperthermia with varying degrees of success. The independent effects of exercise may cloud interpretation of results as it causes increased heat production, fluid shifts and ROS production making isolation of these variable challenging in human models. Although rodent studies are relevant models to answer these questions, the findings may lack translation to humans. Even though current human studies present methodological issues, it is possible that dehydration increases the severity of muscle damage and prolongs recovery, especially in combination with hyperthermia.
What Do We Know?
- Dehydration can negatively affect acute endurance exercise performance, while rehydration has been shown to improve subsequent exercise bouts.
- Some of the physiological perturbations that are responsible for decrements in performance during dehydration such as reduced blood flow to the contracting muscle, alterations in muscle metabolism and impediments in thermoregulation may also contribute to EIMD.
- EIMD is caused by repetitive eccentric muscle contraction resulting in loss of skeletal muscle function and DOMS.
- Independent of dehydration, hyperthermia causes alterations in the skeletal muscle that may increase susceptibility to EIMD.
What More Do We Need to Know?
- The independent effect of dehydration on EIMD is unknown. Well controlled human exercise trials with consistent methodology must be completed before conclusive recommendations can be made.
- While EIMD is primarily the consequence of eccentric skeletal muscle contractions, the effect of hydration status on other types of isolated skeletal muscle contractions (concentric, isometric) is unknown.
- Crucial elements of experimental design that must be standardized in future studies include: the mode of dehydration and EIMD induction; the length of time between dehydration induction, EIMD and subsequent measurements, and the time points at which blood samples are drawn following the intervention; and the need for human skeletal muscle sampling.
- How dehydration modifies EIMD and to what extent, is unknown. However, potential mechanisms may stem from the effects of osmotic stress, hyperthermia and muscle fiber swelling.
- The level of dehydration that is necessary to influence EIMD and prolong recovery has yet to be determined.
- Studies suggest that hydration status is an important variable that should be considered when attempting to minimize EIMD and maximize recovery, although more research needs to be performed.
ACKNOWLEDGEMENTS
The authors would like to thank Dr. Orlando Laitano and Dr. Rebecca Randell for their assistance in review and preparation of the manuscript. Michelle King and Lindsay Baker are employees of the Gatorade Sports Science Institute, a division of PepsiCo, Inc. The views expressed in this article are those of the authors and do not necessarily reflect the position or policy of PepsiCo, Inc.
REFERENCES:
Ahmadizad, S., M.S. El-Sayed. and D.P. MacLaren (2006). Effects of water intake on the responses of haemorheological variables to resistance exercis. Clin. Hemorheol. Microcirc. 35:317-327.
Bourque, C.W. (2008). Central mechanisms of osmosensation and systemic osmoregulation. Nat. Rev. Neurosci. 9:519-531.
Castellani, J., E.J. Zambraski, M. . Sawka, and M.L. Urso (2016). Does high muscle temperature accentuate skeletal muscle injury from eccentric exercise? Physiol. Rep. 4:e12777.
Cheuvront, S.N., and R.W. Kenefick (2014). Dehydration: physiology, assessment, and performance effects. Compr. Physiol. 4:257-285.
Clarkson, P.M., and M.J. Hubal (2002). Exercise-induced muscle damage in humans. Am. J. Phys. Med. Rehabil. 81(Suppl):S52-S69.
Cleary, M.A., L.A. Sweeney, Z.V. Kendrick, and M.R. Sitler (2005). Dehydration and symptoms of delayed-onset muscle soreness in hyperthermic males. J. Athl. Train. 40:288-297.
Cleary, M.A., M.R. Sitler, and Z.V. Kendrick (2006). Dehydration and symptoms of delayed-onset muscle soreness in normothermic men. J. Athl. Train. 41:36-45.
Costill, D.L., R. Coté, and W. Fink (1976). Muscle water and electrolytes following varied levels of dehydration in man. J. Appl. Physiol. 40:6-11.
Ebbeling, C B., and P.M. Clarkson (1989). Exercise-induced muscle damage and adaptation. Sports Med. 7:207-234.
Farhat, F., J.F. Grosset, and F. Canon (2018). Water deprivation decreases strength in fast twitch muscle in contrast to slow twitch muscle in rat. Acta Physiol. 224:e13072.
Faulkner, J.A., S.V. Brooks, and J.A. Opiteck (1993). Injury to skeletal muscle fibers during contractions: conditions of occurrence and prevention. Phys. Ther. 73:911-921.
Fridén, J., P.N. Sfakianos, and A.R. Hargens (1986). Muscle soreness and intramuscular fluid pressure: comparison between eccentric and concentric load. J. Appl. Physiol. 61:2175-2179.
Fridén, J., P.N. Sfakianos, A.R. Hargens, and W.H. Akeson (1988). Residual muscular swelling after repetitive eccentric contractions. J. Orthop. Res. 6:493-498.
Gervais, P., I. Martinez de Maranon, C. Evrard, E. Ferret, and S. Moundanga (2003). Cell volume changes during rapid temperature shifts. J. Biotechnol. 102:269-279.
González-Alonso, J., J.A. Calbet, and B. Nielsen (1998). Muscle blood flow is reduced with dehydration during prolonged exercise in humans. J. Physiol. 513:895-905.
Greiwe, J.S., K.S. Staffey, D.R. Melrose, M.D. Narve, and R.G. Knowlton (1998). Effects of dehydration on isometric muscular strength and endurance. Med. Sci. Sports . 30:284-288.
Hargreaves, M., and M. Febbraio (1998). Limits to exercise performance in the heat. Int. J. Sports Med. 19(Suppl 2):S115-S116.
Haussinger, D., E. Roth, F. Lang, and W. Gerok (1993). Cellular hydration state: an important determinant of protein catabolism in health and disease. Lancet 341:1330-1332.
Hill, D.K. (1972). Resting tension and the form of the twitch of rat skeletal muscle at low temperature. J. Physiol. 22:161-171.
Hillman, A.R., R.V. Vince, L. Taylor, L. McNaughton, N. Mitchell, and J. Siegler (2011). Exercise-induced dehydration with and without environmental heat stress results in increased oxidative stress. Appl. Physiol. Nutr. Metab. 36:698-706.
Hirose, L., K. Nosaka, M. Newton, A. Laveder, M. Kano, J. Peake, and K. Suzuki (2004). Changes in inflammatory mediators following eccentric exercise of the elbow flexors. Exerc. Immunol. Rev. 10:75-90.
Hoffmann, E.K., I.H. Lambert, and S.F. Pedersen (2009). Physiology of cell volume regulation in vertebrates. Physiol. Rev. 89:193-277.
Hubal, M.J., T.C. Chen, P.D. Thompson, and P. M. Clarkson (2008). Inflammatory gene changes associated with the repeated-bout effect. Am. J. Physiol. 294:R1628-R1637.
Hyldahl, R.D., and M.J. Hubal (2014). Lengthening our perspective: morphological, cellular, and molecular responses to eccentric exercise. Muscle Nerve 49:155-170.
King, M.A., T.L. Clanton, and O. Laitano (2016). Hyperthermia, dehydration, and osmotic stress: unconventional sources of exercise-induced reactive oxygen species. Am. J. Physiol. 310:R105-R114.
Kozlowski, S., and B. Saltin (1964). Effect of sweat loss on body fluids. J. Appl. Physiol. 19:1119-1124.
Laitano, O., K.K. Kalsi, J. Pearson, M. Lotlikar, A. Reischak-Oliveira, and J. Gonzalez-Alonso (2012). Effects of graded exercise-induced dehydration and rehydration on circulatory markers of oxidative stress across the resting and exercising human leg. Eur. J. Appl. Physiol. 112:1937-1944.
Laitano, O., L.H. Sheikh, A.J. Mattingly, K.O. Murray, L.F. Ferreira, and T.L. Clanton (2018). Osmolality selectively offsets the impact of hyperthermia on mouse skeletal muscle in vitro. Front. Physiol. 9:1496.
Lieber, R.L. (2010). Skeletal muscle structure, function, and plasticity: The physiological basis of rehabilitation. Lippincott Williams and Wilkins, Baltimore, Maryland, USA.
Lindinger, M.I., M. Leung, K.E. Trajcevski, and T.J. Hawke (2011). Volume regulation in mammalian skeletal muscle: the role of sodium-potassium-chloride cotransporters during exposure to hypertonic solutions. J. Physiol. 589:2887-2899.
Maughan, R.J., S.M. Shirreffs, and J.B. Leiper (2007). Errors in the estimation of hydration status from changes in body mass. J. Sports Sci. 25:797-804.
McCartney, D., B. Desbrow, and C. Irwin (2017). The effect of fluid intake following dehydration on subsequent athletic and cognitive performance: a systematic review and meta-analysis. Sports Med. Open 3:13.
McHugh, M.P., D.A. Connolly, R.G. Eston, I.J. Kremenic, S.J. Nicholas, and G.W. Gleim (1999). The role of passive muscle stiffness in symptoms of exercise-induced muscle damage. Am. J. Sports Med. 27:594-599.
Morgan, D.L., and D.G. Allen (1999). Early events in stretch-induced muscle damage. J. Appl. Physiol. 87:2007-2015.
Morris, J.G., M.E. Nevill, L.H. Boobis, I.A. Macdonald, and C. Williams (2005). Muscle metabolism, temperature, and function during prolonged, intermittent, high-intensity running in air temperatures of 33 degrees and 17 degrees C. Int. J. Sports Med. 26:805-814.
Nosaka, K., and P.M. Clarkson (1995). Muscle damage following repeated bouts of high force eccentric exercise. Med. Sci. Sports Exerc. 27:1263-1269.
Oliver, S.R., V.P. Wright, N. Parinandi, and T.L. Clanton (2008). Thermal tolerance of contractile function in oxidative skeletal muscle: no protection by antioxidants and reduced tolerance with eicosanoid enzyme inhibition. Am. J. Physiol. 295:R1695-R1705.
Owens, D.J., C. Twist, J.N. Cobley, G. Howatson, and G.L. Close (2019). Exercise-induced muscle damage: What is it, what causes it and what are the nutritional solutions? Eur. J. Sport Sci. 19:71-85.
Ozkan, I., and C.H. Ibrahim (2016). Dehydration, skeletal muscle damage and inflammation before the competitions among the elite wrestlers. J. Phys. Ther. Sci. 28:162-168.
Paik, I.Y., M.H. Jeong, H.E. Jin, Y.I. Kim, A.R. Suh, S.Y. Cho, H.T. Roh, C.H. Jin, and S.H. Suh (2009). Fluid replacement following dehydration reduces oxidative stress during recovery. Biochem. Biophys. Res. Commun. 383:103-107.
Sawka, M.N. (1992). Physiological consequences of hypohydration: exercise performance and thermoregulation. Med. Sci. Sports Exerc. 24:657-670.
Seifert, J.G., R.W. Kipp, M. Amann, and O. Gazal (2005). Muscle damage, fluid ingestion, and energy supplementation during recreational alpine skiing. Int. J. Sport Nutr. Exerc. Metab. 15:528-536.
Senay, L.C., Jr., and J.M. Pivarnik (1985). Fluid shifts during exercise. Exerc. Sport Sci. Rev. 13:335-387.
Sjogaard, G. (1983). Electrolytes in slow and fast muscle fibers of humans at rest and with dynamic exercise. Am. J. Physiol. 245:R25-R31.
Sjogaard, G., R.P. Adams, and B. Saltin (1985). Water and ion shifts in skeletal muscle of humans with intense dynamic knee extension. Am. J. Physiol. 248:R190-R196.
Steinbacher, P., and P. Eckl (2015). Impact of oxidative stress on exercising skeletal muscle. Biomolecules 5:356-377.
Tidball, J.G. (2005). Inflammatory processes in muscle injury and repair. Am. J. Physiol. 288:R345-R353.
Toft-Bertelsen, T.L., B.R. Larsen, and N. MacAulay (2018). Sensing and regulation of cell volume - we know so much and yet understand so little: TRPV4 as a sensor of volume changes but possibly without a volume-regulatory role? Channels 12:100-108.
Usher-Smith, J.A., C.L. Huang, and J.A. Fraser (2009). Control of cell volume in skeletal muscle. Biol. Rev. Camb. Philos. Soc. 84:143-159.
Vijayan, K., J.L. Thompson, K.M. Norenberg, R.H. Fitts, and D.A. Riley (2001). Fiber-type susceptibility to eccentric contraction-induced damage of hindlimb-unloaded rat AL muscles. J. Appl. Physiol. 90:770-776.
Warren, G.L., D.A. Lowe, and R.B. Armstrong (1999). Measurement tools used in the study of eccentric contraction-induced injury. Sports Med, 27:43-59.
Yamamoto, L.M., D.A. Judelson, M.J. Farrell, E.C. Lee, LE. Armstrong, D.J. Casa, W.J. Kraemer, J.S. Volek, and C.M. Maresh (2008). Effects of hydration state and resistance exercise on markers of muscle damage. J. Strength Cond. Res. 22:1387-1393.