KEY POINTS
- Carbohydrate (CHO) availability is fundamental for high-intensity endurance performance, contributing over 80% of the total energy expenditure via both muscle glycogen (~60%) and blood glucose (~20%) oxidation when working at intensities of 80% V̇O2max and above.
- The body’s finite CHO stores (~500 g) can sustain high-intensity endurance exercise for ~90 min. Therefore, the body aims to spare this limited CHO supply where possible and utilize fat substrates during submaximal exercise.
- High-fat or ketogenic diets increase the utilization of fat substrates during prolonged, submaximal exercise but impair carbohydrate metabolism. Current evidence demonstrates that high-fat diets impair exercise economy and do not improve high-intensity performance.
- Exogenous ketone drinks increase circulating ketones and reduce lactate production during high-intensity cycling. Preliminary evidence suggests a ketone monoester drink may improve cycling time-trial performance but a diester may impair performance.
- Athletes should periodize CHO intake during training, including sessions with higher and lower CHO availability, to maximize skeletal muscle adaptations and align with the goals of the training session.
- Athletes require CHO before and during competition to fuel the high-intensity, maximal efforts required in racing to support winning performances and recovery.
INTRODUCTION
Prolonged, endurance-based exercise (> 90 min) predominately relies on oxidative metabolism for skeletal muscle contraction, utilizing both carbohydrate (CHO, i.e., muscle and liver glycogen and blood glucose) and fat-based fuels as substrates (i.e., intramuscular triglycerides [IMTG] and blood-borne free fatty acids [FFA]) (Brooks & Mercier, 1994). Following repeated periods of prolonged endurance training, skeletal muscle adaptations result in a slower utilization of CHO substrates and less lactate production, and an increased reliance on fat substrates during low- to moderate-intensity exercise (i.e., 45-65% of V̇O2max) when working at the same absolute (pre-training) exercise intensity (Bergman et al., 1999). In addition to an increased capacity to utilize fat following periods of training, there is also an increase in the capacity to store muscle glycogen and reach higher maximal rates of carbohydrate oxidation.
As a result of skeletal muscle adaptations and the increased utilization of fat-based fuels following training, researchers, practitioners and athletes have been exploring nutritional methods which can “spare” CHO substrates and enhance rates of whole-body fat oxidation to improve exercise capacity (i.e., high-fat and/or ketogenic diets). More recently, researchers and athletes have also investigated an alternative oxidative fuel source to CHO and fat-based fuels, named ketone supplementation, with a similar aim of altering substrate metabolism and improving endurance performance. However, despite the current hype regarding the latter, few studies have investigated ketone supplementation in situations which are realistic of race-like conditions and therefore enable recommendations to athletes to be made.
This Sports Science Exchange article provides a brief overview of the reliance on CHO as a fuel for successful endurance performance and the adaptations that occur with endurance training with special reference to the exercise intensities that athletes train and race at. The above-mentioned topical dietary and supplementation strategies will also be discussed, including changes to skeletal muscle adaptations and substrate metabolism; however, the primary focus will be to assess their efficacy for endurance performance.
ENDURANCE TRAINING PROMOTES ADAPTATIONS AND SHIFTS IN SUBSTRATE UTILIZATION AND INCREASES THE MAXIMAL CAPACITIES FOR FAT AND CHO OXIDATION
It is well established that repeated periods of prolonged endurance training induce skeletal muscle adaptations which lead to a lower respiratory exchange ratio (RER, volume of carbon dioxide produced/volume of oxygen consumed), reduced muscle glycogen utilization, and increased fat oxidation at the same absolute power output/speed as pre-training (Holloszy & Coyle, 1984). The concept of utilizing more fat during exercise and “sparing” CHO has been investigated from as early as 1920 using indirect calorimetry (Krogh & Lindhard, 1920) and ~50 years later using the percutaneous biopsy technique (Bergstrom et al., 1967). However, few studies have investigated whole body rates of substrate oxidation at the same absolute and relative workloads following periods of training.
Bergman et al. (1999) trained participants for nine weeks and measured changes in substrate utilization during cycling at 65% V̇O2max prior to training and at the same absolute (i.e., 65% of pre-training V̇O2max) and relative intensities (65% of post-training V̇O2max). The training program increased V̇O2max by 15% and increased whole-body rates of fat oxidation at the same absolute power output. However, when tested at the same relative intensity, no difference was measured in RER (0.95), FFA uptake or IMTG utilization. Overall fat and CHO utilization was higher (3%) than pre-training because of the higher absolute power output post-training and subsequently greater energy demand. This study demonstrated that although substrate utilization was slightly modified following periods of endurance training, CHO remained the predominant fuel source during moderate-intensity exercise. Bergman and Brooks (1999) further examined the effects of training and nutritional status on substrate utilization during cycling and although differences were measured when cycling at low exercise intensities (i.e., ≤ 40% V̇O2max), when cycling at 60% and 75% V̇O2max, no training or nutrient interaction was identified. Together these findings demonstrated that when training at a moderate intensity, CHO is the predominant fuel source for the working muscle.
ENDURANCE TRAINING AND RACING REQUIRES HIGH RATES OF CHO UTILIZATION
The aim of an athlete’s training is to promote adaptations to enable them to work at high absolute and relative power outputs/speeds for a given time or distance. As such, athletes effectively need to become more, rather than less, reliant on CHO substrates (Hawley, 2002). However, few studies have investigated substrate utilization at realistic race intensities, likely due to the difficulty in recruiting highly trained participants and the complexity of carrying out these studies in laboratory settings. Romijn et al., (1993) were one of the first to show changes in endogenous (muscle) energy contribution during cycling in trained male subjects at different exercise intensities, and this study has now been cited more than 1,500 times. These workers identified that the absolute energy contribution from plasma glucose and FFA did not increase with increasing exercise intensity. Instead, the higher energy demand was met from a greater utilization of intramuscular substrates (i.e., muscle glycogen and IMTG), with muscle glycogen contributing ~70% of the energy expenditure when cycling at 85% V̇O2peak. A follow-up study on trained females supported these findings (Romijn et al., 2000). More recent work in our laboratory identified the substrate demands of intense cycling time-trials, lasting 60, 90 and 120 min at ≥ 80% V̇O2max (Hawley & Leckey, 2015; Torrens et al., 2016) and half-marathon running at ~ 80% V̇O2max (Leckey et al., 2016). The studies included CHO-fed conditions (i.e., CHO loading for 24 h, CHO meal before and CHO feeding during exercise) and the provision of a pharmacological drug (Nicotinic acid) that suppresses the release of FFA from the adipose tissue, to determine the effect on substrate metabolism and performance. The running study also included fasted conditions (i.e., CHO loading but no fueling before or during exercise) to truly test the contribution of fat in conditions which typically drive increased fat utilization (Leckey et al., 2016).
The studies reported an 85-95% energy contribution from CHO-based substrates across the cycling time-trials (up to 120 min) and the half-marathon running (up to 90 min) when in a CHO-fed state and the latter study showed > 80% utilization when fasted (Figure 1). CHO oxidation rates were 4.8, 4.6 and 4.3 g/min when cycling for 60, 90 and 120 min, respectively and were 4.2 g/min when running for ~90 min at ~ 80% V̇O2max in comparison to fat oxidation which ranged from 0.2-0.4 g/min in both studies. While further research is required investigating the metabolic demands of training and racing in elite athletes, the current available research shows that during prolonged, high-intensity exercise, CHO substrates are the predominant fuel sources for the working muscles (Figure 1).
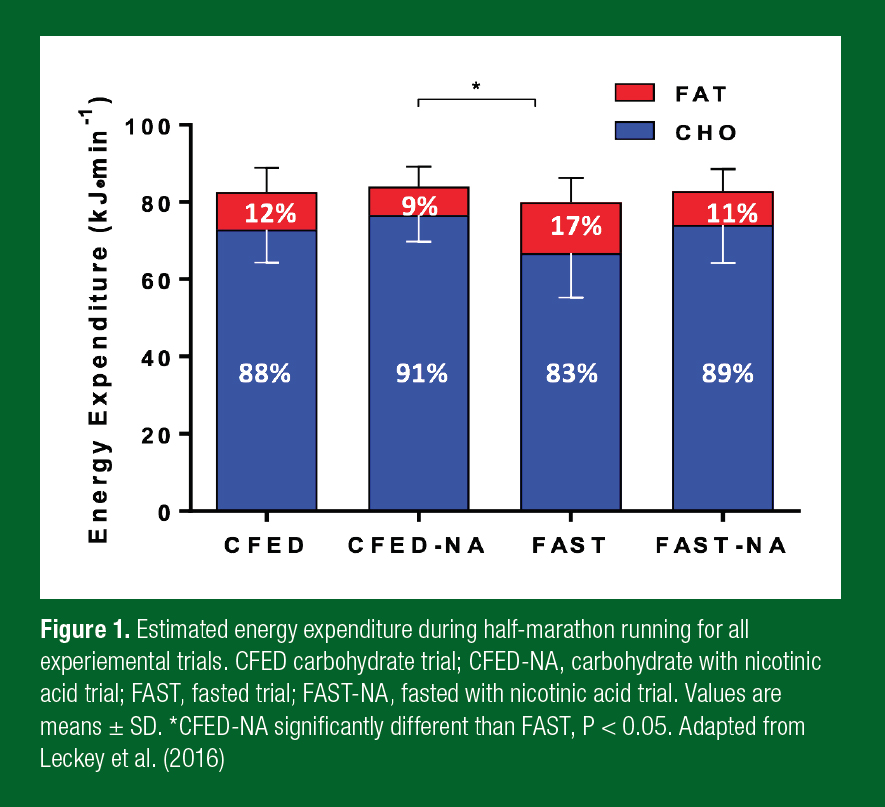
HIGH-FAT DIETS SHIFT SUBSTRATE UTILIZATION BUT MAY IMPAIR PERFORMANCE
The concept of manipulating the proportions of CHO and fat intake to alter fuel utilization during exercise was investigated a century ago. Krogh and Lindhard (1920) reported that RER was lower and perceived effort was higher during submaximal cycling when participants ingested a high-fat, low-CHO diet for three days compared to a CHO-based diet. These workers measured a 5.5% greater energy yield per liter of O2 consumed during submaximal cycling with primarily CHO substrates compared to fat-based fuels. Following this early work and the introduction of the muscle biopsy technique in 1966, a host of studies investigated substrate utilization during endurance exercise and the effects of altering dietary intake on fuel utilization.
However, over the past 40 years research studies have focused on maximizing the contribution of fat to fuel muscular work via the ingestion of a high-fat, low-CHO diet (Noakes et al., 2014; Phinney et al., 1983). The concept of such a dietary practice is that athletes can increase the utilization of the abundant endogenous fat stores (> 30,000 kcal, even in the very lean athlete) and therefore “spare” the limited storage of CHO sources (~2,000 kcal). High-fat dietary strategies range from diets consisting of 65% energy intake from fat (~4 g/kg) and < 20% energy intake from CHO (~2.5 g/kg), to the chronic “ketogenic” diet which consists of < 20 g/d CHO and 80% energy intake from fat (~4.5-5 g/kg). Despite endurance athletes having optimized their skeletal muscle adaptation through prolonged periods of training, high-fat dietary studies have shown that the transport, uptake and oxidation of fat-based fuels can be increased further (Helge, 2002). An overview of the efficacy of high-fat diets for training and endurance racing is discussed below, focusing on only a select few of the vast number of studies available.
Ketogenic Diets and Exercise Performance
Phinney et al. (1983) completed the first modern day high-fat study, providing five well-trained cyclists with seven days of an energy balanced diet (high-CHO; 35-50 kcal/kg/d, 1.75 g protein/kg/day with the remainder of energy coming from CHO [66%] and fat [33%]) followed by 28 days of an isoenergetic, high-fat, low-CHO diet (KETO; < 20 g/day CHO) to investigate the effects of high dietary fat intake on cycling performance and substrate utilization. Following the dietary intervention, participants completed a V̇O2max test and a time to volitional fatigue cycling trial at 63% of V̇O2max to evaluate exercise capacity. Results showed that the V̇O2max was reduced in four participants following the KETO diet and RER at the end of the maximal test decreased from 1.0 to < 0.9, while no difference in exercise capacity was measured between the two dietary conditions (high-CHO, 147 ± 13 min vs. KETO, 151 ± 25 min; P = 0.9). Although the preservation of exercise capacity appears impressive, when individual differences were examined, the performance of two cyclists worsened after the KETO diet, one performed the same and one of the two cyclists who managed to improve their performance actually rode 84 min longer, which markedly skewed the mean time to fatigue. RER during the submaximal cycle was reduced from 0.83 to 0.72 in the KETO condition, therefore successfully increasing rates of fat oxidation. However, an associated three-fold drop in glucose oxidation and a four-fold reduction in muscle glycogen utilization were also measured (Phinney et al., 1983). Although this study provides insights into the effects of manipulating substrate utilization, the chosen exercise intensity (63% V̇O2max) has little to no relevance in relation to the intensities required for elite athletes aiming to win races.
In a more recent study using a similar dietary protocol, Burke et al. (2017) examined the effects of a ketogenic, low-CHO diet on substrate utilization and performance in world-class race-walkers. Twenty-nine race-walkers consumed one of three isoenergetic diets: high carbohydrate diet (HCHO: 8.6 g/kg/d CHO, 2.1 g/kg/d protein and 1.2 g/kg/d fat); periodized diet (PCHO: timing of macronutrient content was altered around training to ensure specific sessions were performed in a low-CHO state); or a low-CHO, high-fat diet (LCHF: < 5.0 g/kg/d CHO, 4.7 g/kg/d fat and 2.2 g/kg/d protein) during three weeks of an intensified training block. Prior to and after the three weeks of dietary and training intervention, participants completed a graded exercise test to measure walking economy, a 25 km standardized walk (i.e., 20 km race pace) to incorporate both laboratory and field testing to assess changes to substrate utilization, and finally, a 10 km International Association of Athletics Federation sanctioned race to measure performance. Following the intervention period, RER during the graded exercise test reduced across four stages of the exercise test in the LCHF condition (RER stages 1-4, 0.86-0.99 to 0.73-0.87). Concomitant with the altered substrate utilization, absolute O2 cost was greater across the four test stages in the LCHF compared to HCHO condition. Findings from the 25 km standardized walk showed increased whole-body rates of fat oxidation from ~0.6 g/min to ~1.6 g/min when walking at 80% V̇O2peak, with the latter being the highest rates of fat oxidation ever to be reported in the literature (Burke et al., 2017). Lastly, despite a training-induced increase in V̇O2peak in all dietary conditions and an increased ability to utilize fat substrates, only the HCHO and PCHO conditions improved their 10 km race-walk time (6.6% and 5.3%, respectively), with no improvement measured in the LCHF condition. This lack of performance improvement measured in the LCHF condition was associated in part with the greater O2 consumption required to sustain the same speed as prior to the intervention (Burke et al., 2017).
High-fat diets, CHO restoration and exercise performance
In addition to “ketogenic” diets saturating the skeletal muscle with fat and therefore challenging the muscle to predominantly utilize fat-based fuels, such diets also present the challenge of training with low-CHO availability (reduced muscle and potentially liver glycogen) (Yeo et al., 2011). Therefore, recent studies investigating the effects of high-fat diets have introduced “nutritional periodization” where a short-term high-fat diet (60-70% energy intake) is consumed for 5-6 days alongside training and is then followed by 24-36 h of high-CHO intake (70-80% energy intake), previously termed “CHO restoration” (Burke et al., 2002; Havemann et al., 2006; Stellingwerff et al., 2006). This concept is thought to maximize the capacity for fat utilization but also optimize endogenous CHO stores.
Studies encompassing this design have shown that short-term high-fat diets increase whole-body fat oxidation during submaximal exercise (~65 V̇O2peak) and reduce the contribution from CHO-based substrates when compared to an isoenergetic high-CHO diet (Burke et al., 2002). This “sparing” of CHO was initially interpreted as a positive adaptation to high-fat diets, but further research has shown that the reduced utilization of muscle glycogen is a consequence of the downregulation of the key enzyme pyruvate dehydrogenase (PDH) that converts pyruvate to acetyl-CoA in the mitochondria, which directly impairs CHO metabolism rather than “sparing” it (Peters et al., 1998; Stellingwerff et al., 2006). Peters et al. (1998) measured a reduction in PDH in its active form (PDHa) at rest following a high-fat diet and a concomitant increase in the enzyme PDH kinase, which converts PDH to its inactive form. The decrease in CHO oxidation following a high-fat diet was partially mediated by decreased insulin and increased FFA concentrations (Peters et al., 2001).
Following one day of CHO restoration which increased muscle glycogen stores and the ingestion of CHO solutions during exercise to prime the system to utilize CHO-based substrates, the robust adaptations from a high-fat diet persisted during prolonged submaximal exercise (Burke et al., 2002; Stellingwerff et al., 2006). To measure changes to PDH activity, Stellingwerff et al. (2006) had participants cycle for 20 min at 70% V̇O2peak followed by a 1 min maximal sprint (150% peak power output) after the ingestion of a high-fat diet (67% energy intake) or an isoenergetic high-CHO diet (~70% energy intake), with one day of CHO restoration. Skeletal muscle biopsy samples were taken at rest, during the first minute of submaximal cycling and after 1 min of all out sprinting to estimate muscle glycogenolysis. Despite similar muscle glycogen content prior to the onset of exercise in the high-fat and high-CHO conditions, PDH activity at rest was 50% lower following high-fat compared to the HCHO condition. Rates of muscle glycogenolysis were lower during submaximal cycling (211 W, 70% V̇O2peak) and 1 min all out sprinting (502 W) following high-fat compared to high-CHO, despite the latter requiring power outputs which would be heavily reliant on muscle glycogen for optimal performance. Maximal PDH activity typically increases to support the high whole-body rates of CHO oxidation required for moderate to high-intensity exercise (> 80% of V̇O2max). Therefore, the persistent downregulation of PDH following high-fat diets, even following CHO restoration, helps explain why a performance improvement has yet to be reported following high-fat dietary intake. In support of the changes in PDH activity reported following a high-fat diet, slower VO2 kinetics have also been measured when transitioning from 20 W to 80% of the estimated lactate threshold following six days of a high-fat (~73% energy intake) compared to high-CHO diet (80% energy intake), highlighting the detrimental effects of high-fat diets for high-intensity work (Raper et al., 2014).
Havemann et al. (2006) directly measured cycling performance using a 100 km laboratory cycling time trial (TT) which included 1 km and 4 km segments, requiring high-intensity sprinting to simulate real-life racing. Prior to exercise, participants ingested either a high-fat diet (68% energy intake) or an isoenergetic high-CHO diet (68% energy intake) for six days followed by one day of CHO restoration. No difference was reported in the overall 100 km TT (high-fat, 146 min, 54 s vs. high-CHO, 153 min, 10 s) or the 4 km sprints, however power output was lower during the 1 km sprints in high-fat compared to high-CHO conditions. The reduction in power output during the 1 km sprints highlights that a high-fat diet may be detrimental to key elements of a successful race performance including surges, breakaways and sprint finishes (Havemann et al., 2006).
KETONE SUPPLEMENTATION ALTERS CIRCULATING METABOLITES AND FUEL UTILIZATION, BUT MAY IMPAIR PERFORMANCE
The adaptations to ingesting high dietary fat for short-term or chronic periods have been thoroughly investigated; however, the effects of the concomitant reduction in CHO intake (i.e., < 50 g/day) have been researched less. In this regard, the production of ketone bodies, which can be utilized as an alternative fuel source by skeletal muscle and the brain is stimulated following periods of low-CHO or high-fat intake. The term “ketone bodies” refers to β-hydroxybutyrate (βHB), acetone and acetoacetate (AcAc), however acetone is mainly lost through expiration and secreted in the urine, whereas AcAc and βHB can be utilized by the brain, heart and skeletal muscle and are therefore often measured in the circulation (Pinckaers et al., 2017).
Endogenous ketone production on circulating ketones
The concentrations of circulating ketone bodies under normal CHO fed conditions are low (i.e., < 0.1 mM) with increases measured following overnight fasting (0.1-0.5 mM), fasted exercise (1.5 mM), prolonged fasting/starvation (~5 days) (~7-8 mM) and chronic exposure to a ketogenic diet (< 0.1 to ~2 mM) (Egan & D'Agostino, 2016; Pinckaers et al., 2017). Although exercising in a fasted state and consuming a high-fat diet increases endogenous ketone production, fasted exercise has little practical relevance for optimal race fueling and the determimental effects of high-fat diets have already been discussed, and therefore the ketone responses to these situations will not be assessed in this article. Instead, ketone bodies derived from exogenous sources may have more practical relevance for athletes as they can avoid exposure to chronic periods of fasting and continue to co-ingest CHO-based fuel sources. Exogenous ketone sources are currently topical within the cycling industry and supplement world, as a potential alternative fuel source for skeletal muscle, with the aim of improving performance.
Exogenous ketone ingestion on circulating ketones, substrate metabolism and exercise performance
Exogenous ketone ingestion is not a novel concept, with ketone salts being investigated since the 1960s (Balasse & Ooms, 1968). However, ketone salts contain a significant salt load (~ 1 g/serving) and are ineffective at rapidly increasing circulating ketones 1-2 h following ingestion. More recently, researchers have measured the response to ketone esters or diesters (Cox & Clarke, 2014; Cox et al., 2016). When ketones are ingested, they undergo metabolism in the liver and are converted to beta hydroxybutyrate (βHB) prior to being oxidized in the skeletal muscle (Cox & Clarke, 2014). Ketone body oxidation appears to be higher in trained individuals compared to untrained, which is potentially associated with higher activities of specific enzymes related to ketone metabolism (Evans et al., 2017; Pinckaers et al., 2017). An increase in ketone body utilization may also impair endogenous CHO availability via reducing liver glucose output and inhibiting PDH, similar to the adaptations observed with a high-fat diet (Beylot et al., 1986; Wicklmayr & Dietze, 1978). As such, ketone bodies may impair exercise capacity since high rates of CHO oxidation are fundamental to intense endurance performance. However, the metabolic response to ketone ingestion is dependent on a host of factors including an individual’s preceding diet, the achieved increase in circulating ketone concentrations, tissue type (i.e., skeletal muscle vs. brain), sex and training status (Pinckaers et al., 2017). This article focuses on dietary intake (i.e., co-ingestion with CHO), changes to circulating ketone concentrations, the skeletal muscle response to ketone ingestion and the effects on performance.
Clarke et al. (2012) produced a ketone monoester, R-3-hydroxybutyl R-3-hydroxybutyrate which has been included in a series of studies examining the safety of the ester, the efficacy in increasing circulating ketone concentrations and the associated effects on metabolism and performance. Clarke & Cox (2015) examined the effect of ketone ester ingestion or a taste-matched placebo in trained rowers, including elite and sub elite, heavy and lightweight, and male and females, prior to completing a 30 min TT in a fasted state. The ketone and placebo drink were provided in the form of a milkshake with dry powder (2.083 g/kg) mixed with water in a 1:1.5 ratio. The drinks were matched for CHO (45%) and protein (20%) and the remaining calories were 5% fat and 30% ketones in the active drink and 35% fat (long chain triglycerides) in the placebo to ensure they were calorie matched.
A performance improvement of 1-2 % was reported following ketone ingestion when compared to the placebo drink (Clarke & Cox, 2015). However, as the circulating ketone response may change when co-ingested with food because of the unique physiological states it presents (Evans et al., 2017; Pinckaers et al., 2017), and athletes do not compete in a fasted state, subsequent studies investigated ketone ingestion with CHO intake. Cox et al. (2016) investigated the effects of ketone ingestion (573 mg/kg, ~337 kcal) with CHO ingestion (40% ketone, 60% CHO) compared to CHO alone on circulating βHB concentration, substrate metabolism and cycling performance. Eight athletes (6 male, 2 females; V̇O2max 5.37, 3.30 L/min, respectively) ingested the first dose (50%) of ketone or CHO 30 min prior to a 60 min bout of steady state cycling (75% maximal workload, 303 or 212 W for males or females, respectively). Following the steady state bout, athletes ingested their second dose of ketone (50% of total drink) or CHO prior to a 30 min TT. During steady state cycling, the ketone plus CHO drink resulted in higher βHB concentration (~1.5 – 2.5 mM vs. 0 mM) and lower FFA and blood lactate concentrations (~3-4 vs. 5-6 mM) compared to the CHO drink, respectively. This circulating ketone concentration was in line with the proposed optimal “nutritional ketosis” range to improve performance (1-3 mM) proposed by Evans et al. (2017). Cox et al. (2016) reported a 2% improvement in TT performance with ketone and CHO ingestion compared to CHO alone, with a greater distance covered (411 ± 162 m, P < 0.05) and lower lactate concentration (~10 vs 14 mM), and sparing the utilization of CHO. This highlights that ketone ingestion improved performance, although as shown in the standard error, a large individual variation was also observed in cycling performance following ketone ingestion.
More recently, our laboratory investigated the effects of a ketone diester on cycling TT performance when in a CHO-fed state (Leckey et al., 2017). A team of 11 male professional cyclists consumed a CHO breakfast with caffeine ingestion 2 h prior to the TT, typical of race day, followed by two doses of either a ketone diester (1,3 butanediol acetoacetate diester, 2 x 250 mg/kg, ~20 mL) or a placebo drink 30 min and immediately prior to starting a 20-min warm-up. Participants then completed a ~31 km TT which was a simulation of the 2017 World Championships course. Ketone diester ingestion resulted in increased βHB (~0.4 vs. 0.2 mM), acetoacetate (~0.4 vs. 0-0.1 mM) and urine ketone concentrations (2-5 vs. 0 mM) compared to the placebo and a lower lactate concentration immediately following the TT (~7 vs. 12 mM). However, ketone diester ingestion also resulted in a 2 ± 1% (58 s) impairment in TT performance when compared to a placebo (average power output = ~340 vs. 350 W, respectively), which was associated with gut discomfort and higher perception of effort. Although gut discomfort is not often discussed with exogenous ketone ingestion, previous studies have reported side effects including nausea, vomiting and diarrhea (Clarke et al. 2012; Clarke & Cox, 2015). Further work is required to explore different dosing strategies and different forms of exogenous ketone ester ingestion to determine if the side effects can be alleviated and confirm if there are advantages to consuming such drinks for performance.
CURRENT SPORT NUTRITION GUIDELINES ON CARBOHYDRATE INTAKE
Sport nutrition guidelines in the 1990s recommended a high intake of CHO-based foods around training sessions and competition with the goal of maximizing muscle glycogen stores and exogenous CHO sources (Coyle, 1991). However, with evolving research these dietary guidelines are regularly updated to incorporate the latest scientific findings and therefore provide optimal, up-to-date advice to athletes and practitioners. As such, recent guidelines no longer include the recommendation of high-CHO for all training sessions and competition; instead, they focus on matching “CHO availability” to the energy demands of the training, taking into considering the duration and intensity of the exercise (Burke et al., 2011; Thomas et al., 2016). Additionally, the guidelines recognize that CHO intake should be individualized depending on training and competition requirements, acknowledging that athletes may selectively choose to complete training sessions with lower endogenous and/or exogenous CHO availability or delay the replenishment of CHO post-training to promote skeletal muscle adaptation and improve performance (Bartlett et al., 2015; Marquet et al., 2016).
SUMMARY AND PRACTICAL APPLICATIONS
Endurance athletes train to promote physiological and metabolic adaptations that enhance or maximize the ability to work at higher absolute and relative power outputs. Although prolonged periods of training induce shifts in substrate utilization, skeletal muscle relies on CHO-based fuels when contracting at moderate to high exercise intensities (≥ 65% V̇O2max). Despite current interest in high-fat, low-CHO diets for endurance athletes, research currently shows no improvement to exercise capacity or performance and instead has identified impaired muscle glycogen utilization. The limited research on exogenous ketone ester ingestion shows ketone drinks can increase circulating ketones and reduce the reliance on CHO-based fuels during submaximal exercise, however further research is required to determine their efficacy for performance and the tolerability for athletes under realistic race conditions. Given the current research, athletes should periodize their CHO intake based on the goal, intensity and duration of a training session in order to maximize skeletal muscle adaptations. However, CHO should be ingested before and during competition to align with athlete goals of maximizing whole body rates of CHO oxidation to maintain exercise intensity and ultimately performance.
References
Balasse, E., and H.A. Ooms (1968). Changes in the concentrations of glucose, free fatty acids, insulin and ketone bodies in the blood during sodium beta-hydroxybutyrate infusions in man. Diabetologica 4: 133-135.
Bartlett, J.D., J.A, Hawley, and J.P. Morton (2015). Carbohydrate availability and exercise training adaptation: too much of a good thing? Eur. J. Sport Sci, 15:3-12.
Bergman, B.C., and G.A. Brooks (1999). Respiratory gas-exchange ratios during graded exercise in fed and fasted trained and untrained men. J. Appl. Physiol. 86:479-487.
Bergman, B.C., G.E, Butterfield, E.E., Wolfel, G.A. Casazza, G.D. Lopaschuk, and G.A. Brooks (1999). Evaluation of exercise and training on muscle lipid metabolism. Am. J. Physiol. 276:E106-E117.
Bergstrom, J., L. Hermansen, E. Hultman, and B. Saltin (1967). Diet, muscle glycogen and physical performance. Acta Physiol. Scand. 71:140-150.
Beylot, M., Y. Khalfallah, J.P. Riou, R. Cohen, S. Normand, and R. Mornex (1986). Effects of ketone bodies on basal and insulin-stimulated glucose utilization in man. J. Clin. Endocrinol. Metab. 63:9-15.
Brooks, G.A., and J. Mercier (1994). Balance of carbohydrate and lipid utilization during exercise: the "crossover" concept. J. Appl. Physiol. 76:2253-2261.
Burke, L.M., J.A, Hawley, D.J. Angus, G.R. Cox, S.A. Clark, N.K. Cummings, B. Desbrow, and M. Hargreaves (2002). Adaptations to short-term high-fat diet persist during exercise despite high carbohydrate availability. Med. Sci. Sports Exerc. 34:83-91.
Burke, L.M., J.A. Hawley, S.H. Wong, and A.E. Jeukendrup (2011). Carbohydrates for training and competition. J. Sports Sci. 29:Suppl 1:S17-S27.
Burke, L.M., M.L. Ross, L.A. Garvican-Lewis, M. Welvaert, I.A. Heikura, S.G. Forbes, J.G. Mirtschin, L.E. Cato, N. Strobel, A.P. Sharma, and J.A. Hawley (2017). Low carbohydrate, high fat diet impairs exercise economy and negates the performance benefit from intensified training in elite race walkers. J. Physiol. 595:2785-2807.
Clarke K., K. Tchabanenko, R. Pawlosky, E. Carter, M.T. King, K. Musa-Veloso, M. Ho, A. Roberts, J. Robertson, T.B. Vanitallie, and R.L. Veech (2012). Kinetics, safety and tolerability of (R)-3-hydroxybutyl (R)-3-hydroxybutyrate in healthy adult subjects. Regul. Toxicol. Pharmacol. 63:401-408, Clarke, K., and P. Cox (2015). Ketone bodies and ketone body esters for maintaining or improving muscle power output. Unpublished observations.
Cox, P.J., and K. Clarke (2014). Acute nutritional ketosis: implications for exercise performance and metabolism. Extreme Physiol. Med. 3:17.
Cox, P.J., T. Kirk, T. Ashmore, K. Willerton, R. Evans, A. Smith, A.J. Murray, B. Stubbs, J. West, S.W. McLure, M.T. King M.S. Dodd, C. Holloway, S. Neubauer, S. Drawer, R.L. Veech, J.L. Griffin, and K. Clarke (2016). Nutritional ketosis alters fuel preference and thereby endurance performance in athletes. Cell Metab. 24:256-268.
Coyle, E.F. (1991). Timing and method of increased carbohydrate intake to cope with heavy training, competition and recovery. J. Sports Sci. 9:29-51; discussion, 51-52.
Egan, B., and D.P. D'Agostino (2016). Fueling performance: Ketones enter the mix. Cell Metab. 24:373-375.
Evans, M., K.E. Cogan, and B. Egan (2017). Metabolism of ketone bodies during exercise and training: physiological basis for exogenous supplementation. J. Physiol. 595:2857-2871.
Havemann, L., S.J. West, J.H. Goedecke, I.A. Macdonald, A. St Clair Gibson, T.D. Noakes, and E.V. Lambert. (2006). Fat adaptation followed by carbohydrate loading compromises high-intensity sprint performance. J. Appl. Physiol. 100:194-202.
Hawley, J.A. (2002). Adaptations of skeletal muscle to prolonged, intense endurance training. Clin. Exp. Pharmacol. Physiol. 29:218-222.
Hawley, J.A., and J.J. Leckey (2015). Carbohydrate dependence during prolonged, intense endurance exercise. Sports Med. 45(Suppl 1):S5-S12.
Helge, J.W. (2002). Long-term fat diet adaptation effects on performance, training capacity, and fat utilization. Med. Sci. Sports Exerc. 34:1499-1504.
Holloszy, J.O., and E.F. Coyle (1984). Adaptations of skeletal muscle to endurance exercise and their metabolic consequences. J. Appl. Physiol. 56:831-838.
Krogh, A., and J. Lindhard (1920). The relative value of fat and carbohydrate as sources of muscular energy: with appendices on the correlation between standard metabolism and the respiratory quotient during rest and work. Biochem. J. 14:290-363.
Leckey, J.J., L.M. Burke, J.P. Morton, and J.A. Hawley (2016). Altering fatty acid availability does not impair prolonged, continuous running to fatigue: evidence for carbohydrate dependence. J. Appl. Physiol. 120:107-113.
Leckey, J.J., M.L. Ross, M. Quod, J.A, Hawley and L.M. Burke (2017). Ketone diester ingestion impairs time-trial performance in professional cyclists. Front. Physiol. 8:806.
Marquet, L.A., J. Brisswalter, J. Louis, E. Tiollier, L.M. Burke, J.A. Hawley, and C. Hausswirth (2016). Enhanced endurance performance by periodization of carbohydrate intake: "sleep low" strategy. Med. Sci. Sports Exerc. 48:663-672.
Noakes, T., J.S. Volek, and S.D. Phinney (2014). Low-carbohydrate diets for athletes: what evidence? Br. J. Sports Med. 48:1077-1078.
Peters, S.J., R.A. Harris, P. Wu, T.L. Pehleman, G.J. Heigenhauser, and L.L. Spriet (2001). Human skeletal muscle PDH kinase activity and isoform expression during a 3-day high-fat/low-carbohydrate diet. Am. J. Physiol. 281:E1151-E1158.
Peters, S.J., T.A. St Amand, R.A. Howlett, G.J. Heigenhauser, and L.L. Spriet (1998). Human skeletal muscle pyruvate dehydrogenase kinase activity increases after a low-carbohydrate diet. Am. J. Physiol. 275:E980-E986.
Phinney, S.D., B.R. Bistrian, R.R. Wolfe, and G.L. Blackburn (1983). The human metabolic response to chronic ketosis without caloric restriction: physical and biochemical adaptation. Metabolism 32: 757-768.
Pinckaers, P.J., T.A. Churchward-Venne, D. Bailey, L.J. van Loon (2017). Ketone bodies and exercise performance: the next magic bullet or merely hype? Sports Med. 47:383-391.
Raper, J.A., L.K. Love, D.H. Paterson, S.J. Peters, G.J. Heigenhauser, and J.M. Kowalchuk (2014). Effect of high-fat and high-carbohydrate diets on pulmonary O2 uptake kinetics during the transition to moderate-intensity exercise. J. Appl. Physiol. 117:1371-1379.
Romijn, J.A., E.F. Coyle, L.S. Sidossis, A. Gastaldelli, J.F. Horowitz, E. Endert, and R.R. Wolfe (1993). Regulation of endogenous fat and carbohydrate metabolism in relation to exercise intensity and duration. Am. J. Physiol. 265:E380-E391.
Romijn, J.A., E.F. Coyle, L.S. Sidossis, J. Rosenblatt, and R.R. Wolfe (2000). Substrate metabolism during exercise intensities bin endurance trained women. J. Appl. Physiol. 88:1707-1714.
Stellingwerff, T., L.L. Spriet, M.J. Watt, N.E. Kimber, M. Hargreaves, J.A. Hawley, and L.M. Burke (2006). Decreased PDH activation and glycogenolysis during exercise following fat adaptation with carbohydrate restoration. Am. J. Physiol. 290:E380-E388.
Thomas, D.T., K.A. Erdman, and L.M. Burke (2016). American College of Sports Medicine Joint Position Statement. Nutrition and Athletic Performance. Med. Sci. Sports Exerc. 48:543-568.
Torrens, S.L., J.L. Areta, E.B. Parr, and J.A. Hawley (2016). Carbohydrate dependence during prolonged simulated cycling time trials. Eur. J. Appl. Physiol. 116:781-790.
Wicklmayr, M., and G. Dietze 3rd (1978). Effect of continuously increasing concentrations of plasma ketone bodies on the uptake and oxidation of glucose by muscle in man. Eur. J. Clin. Invest. 8:415-421.
Yeo, W.K., A.L. Carey, L. Burke, L.L. Spriet, and J.A. Hawley (2011). Fat adaptation in well-trained athletes: effects on cell metabolism. Appl. Physiol. Nutr. Metab. 36:12-22.