KEY POINTS
- There has been considerable interest recently in the concept of sweat biomarkers, which is generally defined as the use of sweat as a non-invasive alternative to blood analysis to provide insights into human physiology, health, and performance.
- However, application of sweat diagnostics in sports science has been limited to date. Correlations between sweat and blood have not been established for most constituents. Sweat electrolyte concentrations are not a valid biomarker for real-time assessment of hydration status (i.e., fluid balance) per se.
- Using sweat as a biomarker is challenging because sweat composition is not only influenced by extracellular solute concentrations, but also mechanisms of secretion and/or reabsorption, sweat flow rate, byproducts of sweat gland metabolism, skin surface contamination, and sebum secretions, as well as methodological and inter-individual factors.
- Well-controlled, adequately powered studies are needed to determine the relation between sweat and blood solute concentrations, which will help inform the potential utility of sweat diagnostics as a non-invasive tool for real-time hydration, nutrition, and physiological monitoring in athletes.
INTRODUCTION
The primary purpose of sweating is thermoregulation, as evaporation of sweat from the skin surface is the most effective means to liberate heat from the body during exercise. While sweat is largely salt (sodium chloride, NaCl) water, it also contains many other dissolved solutes, such as vitamins, trace minerals, metabolites, cytokines, and other compounds. The types of solutes in sweat are comparable to the blood (albeit at different concentrations) because extracellular fluid serves as the precursor fluid to primary sweat that is initially secreted into the eccrine glands (Cage & Dobson, 1965). As a result, there is significant interest in the utility of sweat beyond its role in body temperature regulation. In the past ~5 years there has been a surge in research investigating the potential of sweat to serve as a diagnostic tool or biomarker – i.e., a non-invasive alternative to blood analysis to provide insights into human physiology, health, or performance.
Much of the recent research on sweat biomarkers has been driven by technological advances in materials, mechanics, and microsystem designs, thus enabling in situ collection and analyses of sweat chemistry. However, the concept and practice of sweat diagnostics is not new. Perhaps the best example of a sweat biomarker is the association between high sweat chloride concentration and cystic fibrosis, which was first recognized nearly seven decades ago (Di Sant'Agnese et al., 1953). However, apart from the use of sweat chloride to diagnose cystic fibrosis, the application of sweat biomarkers has been limited to date. This is because several questions about mechanisms of sweat solute secretion and methodological challenges remain. Therefore, the purpose of this Sports Science Exchange article is to review: 1) basic mechanisms determining sweat composition; and 2) evidence for the use of sweat as a proxy for blood solute concentrations. The aim is to help elucidate which, if any, sweat constituents have potential as a biomarker for real-time hydration and nutrient status and physiological monitoring in athletes, identify gaps in the literature, and inform the direction of future research.
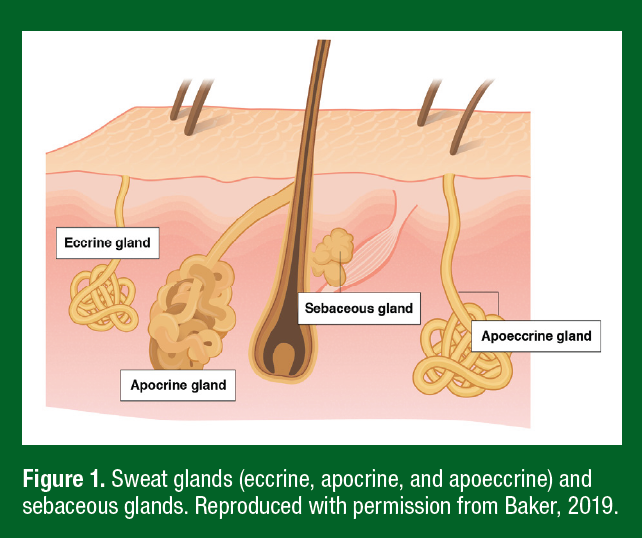
SWEAT GLAND TYPES, STRUCTURE, AND FUNCTION
Sweat glands are classified into three main types: eccrine, apocrine, and apoeccrine (Figure 1). Eccrine glands secrete an aqueous solution comprised mostly of NaCl and are the most ubiquitous gland type (~2–4 million across most of the body surface). Apocrine glands are limited primarily to the axilla, breasts, face, and scalp and produce viscous, lipid-rich sweat containing proteins, sugars, and ammonia (Montagna & Parakkal, 1974a). Apoeccrine glands produce salt-water secretions but are only found in the axillary region. While not a sweat gland, sebaceous glands are present over much of the body surface, particularly the scalp, forehead, and face, where they secrete a viscous, lipid-rich fluid (Montagna & Parakkal, 1974b). Eccrine gland secretions are generally the most relevant to the sweat biomarker discussion since most studies measure sweat from the arms, chest, back, or legs. Still, some studies collect sweat from the face/forehead and therefore secretions from apocrine and sebaceous glands would also contribute to sweat composition. Both sebaceous and apocrine glands are under hormonal control and their secretions are thought to function as pheromones. Sebum may also have antibacterial and antifungal properties (Strauss et al., 1983).
The anatomical structure of the eccrine sweat gland consists of two main functional components: a secretory coil and a duct, both made up of a simple tubular epithelium (Figure 2). During exercise, a large amount of heat is produced by the contracting muscles as a byproduct of metabolism. The resultant increase in body temperature is sensed by central and skin thermoreceptors, which subsequently stimulate the onset of eccrine sweating primarily via sympathetic cholinergic system. Eccrine sweat glands also respond to exercise-related stimuli such as central command, the exercise pressor reflex, osmoreceptors, and possibly baroreceptors (Shibasaki & Crandall, 2010). Upon stimulation, clear cells of the secretory coil secrete primary sweat, which is nearly isotonic to blood plasma with respect to Na+, Cl-, and potassium (K+) (Costill, 1977). The primary function of the eccrine duct is reabsorption of Na+ and Cl− ions, resulting in a hypotonic final sweat excreted onto the skin surface (Sato, 1977) (Figure 2).
OVERVIEW OF SWEAT COMPOSITION
The approximate concentrations of some of the more commonly researched sweat constituents are shown in Figure 3. Although sweat contains a mixture of many solutes, Na+ and Cl− are by far the most concentrated, ranging from 10 to 90 mmol/L (Barnes et al., 2019). Substances present at lower millimolar concentrations include lactate, urea, ammonia, bicarbonate, and potassium. Most other constituents are measured on a micromolar (calcium, magnesium, iron, zinc, copper, ascorbic acid, glucose, uric acid, and individual amino acids) or even smaller scale (nanomolar: thiamine, cortisol; picomolar: cytokines). Approximations for corresponding blood plasma concentrations are also shown in Figure 3. Few constituents have similar concentrations in sweat and blood (e.g. K+). Some solutes are relatively dilute in sweat because of reabsorption in the duct (Na+, Cl−, bicarbonate) or limitations in transport across/between cells of the eccrine gland (e.g., ascorbic acid, glucose, cytokines, cortisol, uric acid). When solutes appear in final sweat at higher concentrations than that of blood, the additional solute could be derived from the skin (e.g., urea, ammonia, amino acids, some trace minerals) or the eccrine sweat glands (e.g., lactate, urea, ammonia). The next section will describe physiological mechanisms and other factors that determine the constituent concentrations measured in final sweat.
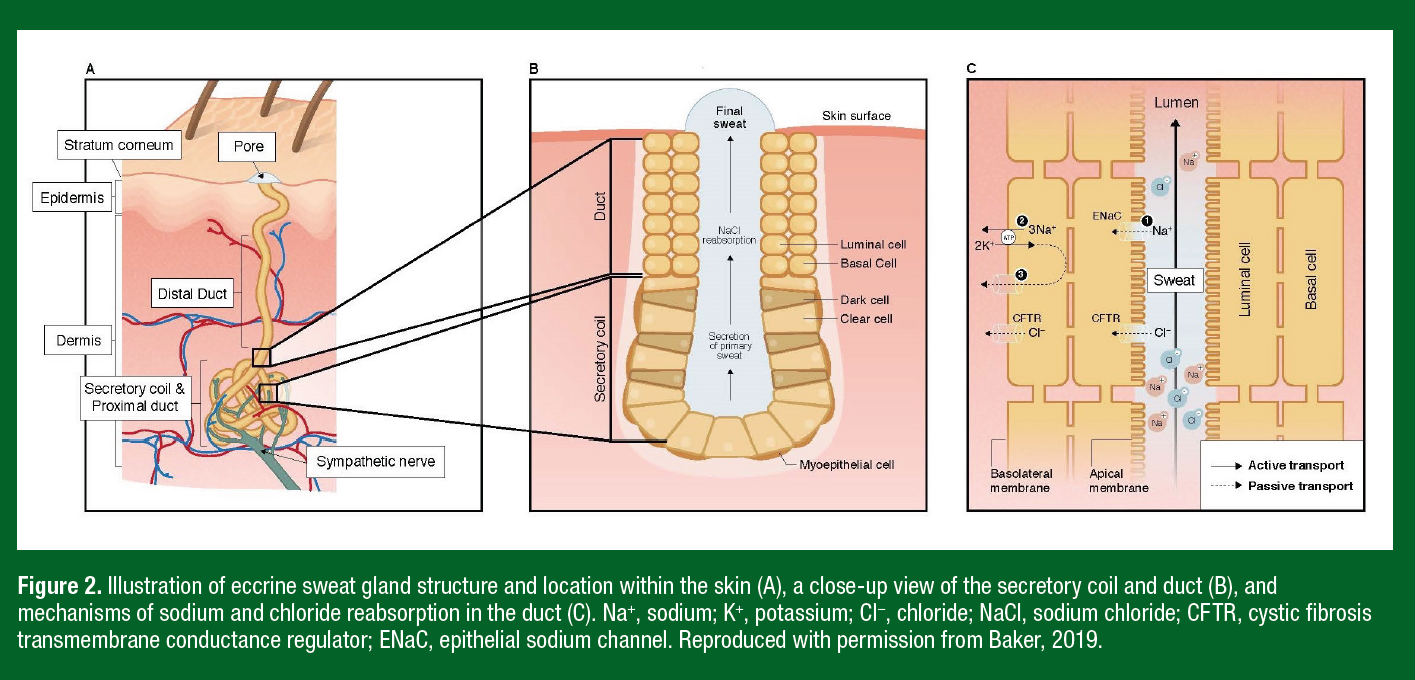
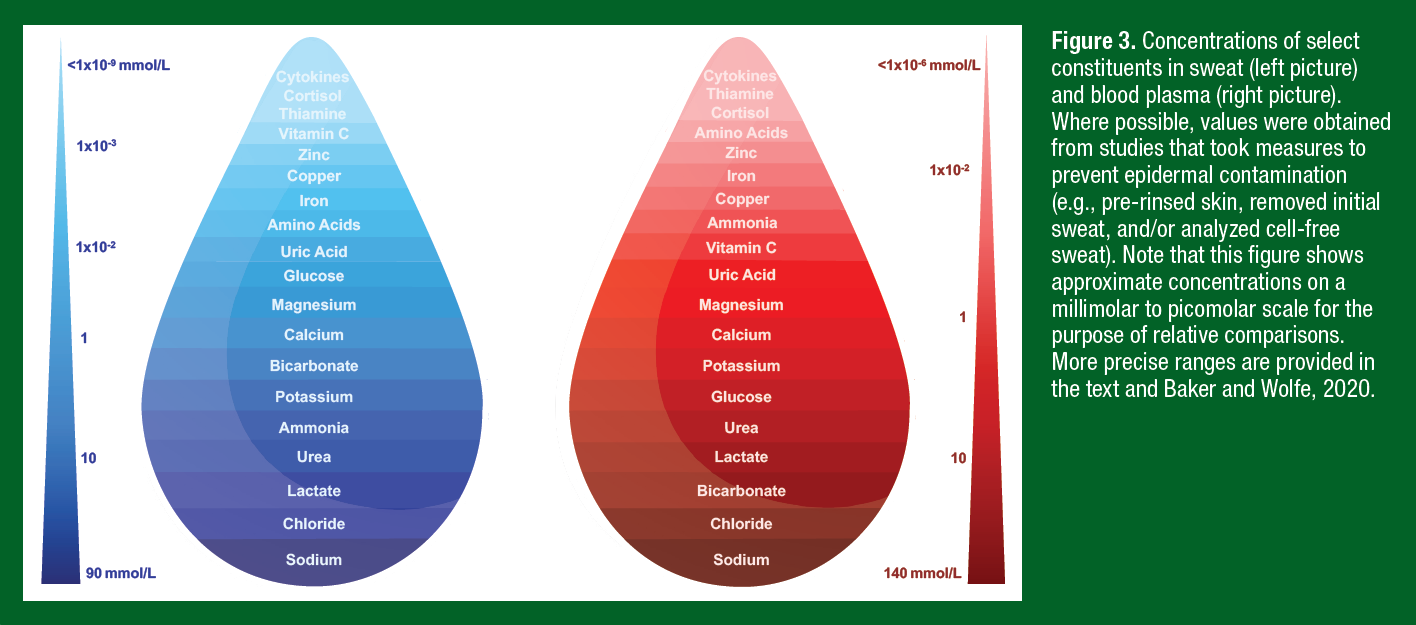
REVIEW OF THE LITERATURE
Sodium, Chloride, and Potassium
Mechanisms. The [Na+] and [Cl−] of final sweat are determined by the rate of ion reabsorption in the duct relative to the rate of ion secretion in the clear cells of the secretory coil. Na+, Cl−, and K+ are secreted into the secretory coil via the Na+-K+-2Cl− cotransport model. Reabsorption of Na+ and Cl− in the duct involves passive influx of Na+ through epithelial Na+ channels (ENaC) of the luminal cells, followed by active transport of Na+ (via Na+-K+-ATPase) through the basal cells of the eccrine duct (Sato, 1977). Cl− reabsorption is largely passive via movement through cystic fibrosis transmembrane conductance regulator (CFTR) channels of both the luminal and basal cells (Figure 2). Na+-K+-ATPase activity and CFTR channel abundance play important roles in determining final sweat [Na+] and [Cl−]. Na+-K+-ATPase activity is influenced by hormonal control of circulating aldosterone (Sato & Dobson, 1970). Resting (genomic) plasma aldosterone concentration is influenced by heat acclimation, fitness, and diet, while non-genomic factors (e.g., exercise and dehydration) stimulate acute changes in circulating aldosterone (Yoshida et al., 2006). CFTR availability is reduced with defects in CFTR genes (cystic fibrosis) (Quinton, 1999) and there is evidence that healthy individuals with salty sweat may also exhibit lower abundance of CFTR channels (Brown et al., 2011). The rate of Na+ and Cl- reabsorption is also flow dependent, such that there is a direct relation between sweating rate and final sweat [Na+] and [Cl-]. As sweating rate increases the rate of Na+ and Cl- secretion in primary sweat increases proportionally more than the rate of Na+ and Cl- reabsorption along the duct, and therefore leads to higher final sweat [Na+] and [Cl-] (Buono et al., 2008).
Evidence as a Biomarker. Because of the multitude of factors impacting ductal Na+ and Cl- reabsorption rates, sweat electrolyte concentrations can vary considerably within and between athletes. Therefore, sweat testing is recommended to determine individualized sweating rates and sweat electrolyte concentrations so that athletes can follow personalized fluid replacement strategies. Several valid methods for sweat testing have been previously described, ranging from laboratory-based to more practical field methods (Baker SSE #161). Sweat testing in this traditional sense helps determine an athlete’s risk for significant fluid and electrolyte losses. However, the utility of sweat [Na+], [Cl−], and [K+] as a biomarker per se in sports applications is questionable. This is due in part to the lack of correlation between sweat and blood [Na+], [Cl−], and [K+] (Klous, de Ruiter, et al., 2021). Even though primary sweat is isotonic with the blood, the rate of reabsorption of Na+ and Cl− in the eccrine duct is independent of plasma electrolyte concentrations. For example, acute increases in exercise intensity alone can result in significant increases in final sweat [Na+] (Buono et al., 2008) without a change in plasma [Na+] (Klous, de Ruiter, et al., 2021). Furthermore, genomic factors impacting resting plasma aldosterone play a more important role in determining final sweat [Na+] than plasma [Na+] (McCubbin et al., 2019; Yoshida et al., 2006).
It has been suggested that sweat electrolyte concentrations can be used as a biomarker to detect dehydration. However, as discussed above, sweat [Na+] and [Cl-] can vary substantially and a change in hydration status is only one of many factors that may play a small role in this variability. Sweat [Na+], [Cl−], and [K+] have been shown to increase (Morgan et al., 2004), decrease (Armstrong et al., 1985), or not change (Walsh et al., 1994) in response to dehydration during exercise and/or heat stress. Furthermore, studies have demonstrated significant changes in sweat [Na+], [Cl−], and/or [K+] in response to variations in exercise intensity (Baker et al., 2019), environment (Dziedzic et al., 2014), or diet (McCubbin et al., 2019) despite limited changes in hydration status during exercise. Taken together, while sweat testing is useful for quantifying NaCl losses during exercise, sweat electrolyte concentrations are not valid biomarkers of hydration status (i.e., fluid balance) per se.
Trace Minerals and Vitamins
Mechanisms. Few studies have investigated mechanisms of sweat secretion for micronutrients other than Na+, Cl−, and K+. Furthermore, measurement of certain minerals in sweat is often confounded by skin surface contamination, as cell-rich sweat calcium (Ca2+), iron (Fe2+), magnesium (Mg2+), and zinc (Zn2+) concentrations are ≥ 2-fold higher than that of cell-poor sweat (Baker & Wolfe, 2020). Studies that have taken measures to collect cell-poor sweat suggest that final sweat trace elements and vitamin concentrations are similar to or less than blood plasma concentrations (Figure 3). The secretion of some elements in primary sweat is influenced by how bound versus free fractions of minerals cross the lipid bilayer membrane of clear cells in the secretory coil of the eccrine gland. Charged ions such as free Ca2+ and Mg2+ are hydrophilic but small, so they may be secreted readily via a paracellular route. By contrast, protein-bound fractions of these elements may not be as readily available for secretion or may occur at a slower rate. This may explain why [Ca2+] and [Mg2+] of cell-poor sweat are more similar to ionized than to total plasma [Ca2+] and [Mg2+] concentrations (Gibinski et al., 1974). The vitamins ascorbic acid and thiamine are large polar molecules and therefore may enter the secretory coil through a paracellular route, but more research is needed to elucidate secretion mechanisms.
Evidence as a Biomarker. There is interest in the potential of wearable sweat sensors for non-invasive measurement of micronutrient status for personalized nutrition, which could be useful in detecting nutritional deficiencies/insufficiencies and supporting dietary behavior change. However, few well-controlled, adequately powered studies have compared trace mineral and vitamin concentrations in sweat versus blood. (Mickelsen & Keys, 1943; Vellar, 1968; Zhao et al., 2021). Interestingly, the only significant correlation reported for trace minerals was between cell-free sweat [Fe2+] and serum [Fe2+], albeit the correlation coefficient was small (r = 0.37) (Vellar 1968a). Some have found a significant change in sweat [Fe2+] and [Zn2+] associated with dietary intake (Milne et al., 1983; Prasad et al., 1963), but these studies included patient populations with known mineral deficiencies or involved controlled interventions designed to deplete and subsequently replete mineral stores of healthy subjects. It is unclear if sweat tests would be sensitive enough to detect smaller fluctuations in micronutrient status. Preliminary data collected with novel wearable biosensors suggest that sweat ascorbic acid, Zn2+, Ca2+, and Fe2+ concentrations increase after oral supplementation in healthy individuals (Kim et al., 2022; Zhao et al., 2021). However, the results suggest a general trend of increased concentrations rather than a linear relation with intake (Kim et al., 2022). Oral administration of ascorbic acid, Zn2+, Ca2+, and Fe2+ yield large initial peaks in sweat concentrations (1-2 hours after intake), followed by gradual decreases in sweat concentrations (Kim et al., 2022; Zhao et al., 2021). More work is needed to determine the utility of sweat as a biomarker for trace mineral and vitamin status.
Metabolites
Mechanisms. Sweat secretion and Na+ reabsorption are active processes and the main avenue of energy production for sweat gland function is oxidative phosphorylation of plasma glucose and glycogen (Sato, 1977; Sato & Dobson, 1973). Thus, byproducts of sweat gland metabolism can have an impact on final sweat composition. For example, there is general agreement that at least some of the lactate in sweat originates from lactate production by the eccrine gland. Subsequently lactate may be transported out of clear cells via monocarboxylate transport proteins (Baker & Wolfe, 2020). Sweat lactate concentration (5-40 mmol/L) typically exceeds that of blood lactate (0.5-2 mmol/L at rest, up to 15-25 mmol/L during exercise), especially at the onset of initial sweating or when flow rates are low. Sweat lactate concentration decreases as sweating rate increases, possibly because of dilution (Buono et al., 2010).
While glucose is present in sweat, the exact mechanisms of secretion are unclear. Most studies suggest that blood glucose is the primary source of sweat glucose. However, glucose concentrations in sweat are 100 times lower than blood (Boysen et al., 1984), likely because its large size and polarity limit the passage of glucose into the lumen of the eccrine gland. For example, some glucose secretion may occur paracellularly, but tight junctions limit large molecule transport. Transcellular movement of glucose into sweat may occur via glucose transporters (GLUT2, and sodium-dependent glucose transporters SGLT3 and SGLT4) (Baker & Wolfe, 2020), but more research is needed to elucidate mechanisms.
As many as 26 different amino acids have been found in sweat on the skin surface, but concentrations can be ≥3x that of blood (Dunstan et al., 2016). Some of the sweat amino acid content may originate from the plasma, with transfer into the secretory coil influenced by molecular weight, polarity, or ligand binding, among other factors (Baker & Wolfe, 2020; Gitlitz et al., 1974). The non-plasma source of amino acids in sweat is likely skin natural moisturizing factors (NMFs) and epidermal proteins (Mark & Harding, 2013). In support of this, several studies have reported a decline in sweat amino acid concentration as exercise duration or sweating rate increase, suggesting possible flushing of skin NMFs or dilution of amino acid concentration occurring with greater sweat volumes (Dunstan et al., 2016; Gitlitz et al., 1974).
Bicarbonate is present in most body fluids and plays an important role in acid–base balance. Secretory mechanisms are unclear but may involve bicarbonate/Cl- channels (Best2) in the dark cells and bicarbonate/Cl- exchangers in luminal cells of the secretory coil (Cui & Schlessinger, 2015; Quinton & Reddy, 1989). Sweat bicarbonate (0.5-5 mmol/L) is lower than concentrations in the blood (22-29 mmol/L). This is because bicarbonate is reabsorbed in the eccrine duct, possibly via CFTR combined with H+ secretion and bicarbonate/Cl- exchangers in luminal cells, which also leads to acidification of final sweat (Choi et al., 2001). As such, the rate of bicarbonate secretion and reabsorption in the eccrine gland determines the pH of final sweat. In addition, much like Na+ and Cl−, bicarbonate reabsorption is inversely related to sweating rate. Therefore, lower sweat flow rates are associated with a lower bicarbonate concentration and lower pH of final sweat (Kaiser et al., 1974).
Evidence as a Biomarker. There is significant interest in sweat lactate and other metabolites in sweat as a more practical and non-invasive alternative to blood sampling. For example, plasma lactate concentration is often used as a biomarker to help determine anaerobic threshold, which is used as an important metric to monitor training status and design workout regimens for athletic performance. However, there is currently little to no evidence of a correlation between sweat and blood metabolites, including lactate, glucose, amino acids, and bicarbonate, in healthy individuals at rest or during exercise (Baker & Wolfe, 2020; Buono et al., 2010; Klous, de Ruiter, et al., 2021). For example, an increase in blood lactate concentration during exercise has been associated with a decrease (Ament et al., 1997; Klous, de Ruiter, et al., 2021) or no change (Alvear-Ordenes et al., 2005; Green et al., 2000) in corresponding sweat lactate concentration.
Some studies did not report correlation results but observed the change in sweat metabolite concentration after manipulation of blood concentrations via substrate ingestion. Exercise-induced sweat bicarbonate concentration did not differ after ingestion of sodium bicarbonate compared with placebo, but sweat pH was significantly higher in the sodium bicarbonate ingestion trial (Patterson et al., 2002). Significant increases in sweat glucose concentrations were observed in response to infusion and ingestion of a glucose bolus, which caused an increase in blood glucose from 60 to 360 mg/dl (Boysen et al., 1984). However, it is unclear if smaller changes in blood glucose would elicit measurable concomitant changes in sweat glucose.
The lack of correlation between sweat and blood metabolite concentrations is perhaps not surprising given the known effects of reabsorption in the duct (bicarbonate), limitations in transport (glucose), and other factors (skin-derived amino acids or gland-derived lactate) discussed previously. While some preliminary data collected with novel wearable devices have reported significant correlations between sweat and blood for glucose and lactate, interpretation of results is difficult due in part to methodological issues, low sample size, or unclear/incomplete analysis and reporting of results (for review see Baker & Wolfe, 2020). It has been suggested that incorporating algorithms to calibrate for individual differences and account for blood-sweat time lag, among other factors, may help improve the correlations. However, well-designed clinical trials are needed to support these hypotheses. Until then, the utility of sweat analysis as a proxy for blood metabolite monitoring remains questionable.
Nitrogenous Wastes
Mechanisms. Nitrogenous wastes such as urea, uric acid, and ammonia are end-products of nitrogen metabolism in the liver. Removal of nitrogenous waste is critical for proper body function and occurs primarily through urination. The principal compound formed from the oxidation of protein is urea, which is a small polar molecule that easily crosses the glandular wall and cell membrane (Komives et al., 1966). Reported sweat urea concentrations (4-12 mmol/L) are often higher than that of plasma (2.5-7 mmol/L), suggesting possible contributions from non-plasma sources. The increased urea in sweat is hypothesized to originate from urea in the epidermis (Brusilow, 1967) or urea production stemming from the split of arginine to ornithine and urea via arginase activity in the eccrine gland or skin (Sato et al., 1989). Indeed, research has shown that sweat-to-blood urea ratios decrease to near unity after profuse sweating, which is thought to flush away metabolites such as urea that originate from the skin and gland itself (Komives et al., 1966). Recent evidence of urea transporter (subtypes UT-A1 and UT-B1) expression in the secretory coil and duct of the eccrine gland suggests there may also be an active mechanism of urea excretion through sweat, possibly accounting for increased sweat urea with end-stage renal disease (Xie et al., 2017). Uric acid is a byproduct of purine metabolism, which is related to adenosine triphosphate (ATP) turnover and degradation during exercise. While uric acid appears in sweat, mechanisms of secretion into the eccrine gland are unknown. Studies have shown that sweat uric acid concentrations are ~10 times lower than that of blood (Huang et al., 2002; Yang et al., 2020). This is possibly because of limited transport across cellular membranes owing to uric acid’s large molecular size.
Ammonia is a waste formed mostly through bacteria in the intestines during protein digestion and used as substrate in the urea cycle. Like urea, ammonia is a small polar molecule, and its secretion may occur through passive diffusion via transcellular or paracellular transport. In addition, ammonia is typically found in higher concentrations in sweat (1-8 mmol/L) than in blood (0.01-0.03 mmol/L). While exact underlying mechanisms for the elevated concentrations are unclear it has been suggested that final sweat could be impacted by ammonia production within the eccrine gland via the breakdown of urea and/or ammonia formation in the skin (Brusilow & Gordes, 1968; Nose et al., 2005), as well as the plasma ammonia (Czarnowski et al., 1992).
Evidence as a Biomarker. Blood ammonia concentration increases with physical strain and muscular activity and elevations in urea are associated with protein catabolism. Therefore, it is thought that sweat ammonia and urea may be useful markers of exercise-related metabolic stress. Pharmacologically-induced sweat ammonia concentration has been shown to increase, concomitant with elevated blood ammonia concentrations, after oral ingestion of ammonium chloride (Czarnowski et al., 1992). However, in an exercise study, increased blood ammonia concentration resulting from increased work rates led to a significant decrease in sweat ammonia concentration (Ament et al., 1997). Moreover, there is limited evidence of a correlation between sweat and blood ammonia, urea, or uric acid concentrations (Yang et al., 2020), especially in a context relevant to healthy athletes (Huang et al., 2002; Klous, de Ruiter, et al., 2021). One study did find significant sweat-blood correlations when using exercise (rugby match) and sauna bathing (pre- and post-match) to stimulate sweating, but ammonia and urea concentrations in sweat only accounted for 7% and 45% of the variation in respective blood concentrations (Alvear-Ordenes et al., 2005). More well-controlled correlation studies in a sports context are needed.
It has been suggested that thermal sweating may serve as a useful avenue to excrete uric acid and urea in times of increased production and elevated serum concentrations. Sweat uric acid concentrations have been higher in gout patients versus healthy subjects (Yang et al., 2020). However, total sweat uric acid losses in healthy subjects, including after prolonged exercise-heat stress, are very low (< 5-10%) compared with the amount typically excreted via urination (Huang et al., 2002).
Stress and Immune Markers
Mechanisms. Cortisol acts as the main glucocorticoid hormone produced by the adrenal cortex and is released in response to psychological and physiological stimuli. Approximately 90% of the endogenous hormone is bound to carriers, while the remaining 5–10% is free cortisol. Unbound cortisol is thought to diffuse readily into cells due to their lipid-rich cell membrane. Thus, it follows that passive diffusion is likely the mechanism by which free cortisol is secreted into the eccrine gland. This may also explain why cortisol concentration in sweat is ~100 times lower than that of blood, as the bound fractions of cortisol are not secreted as readily as free cortisol (Jenkins et al., 1969).
Cytokines have pleiotropic effects crucial to endocrine, autocrine, and paracrine cell signaling related to inflammation, immune response, and infection. Their large molecular weight would seem to preclude secretion into eccrine sweat; however, several studies have detected interleukin (IL)-1α or IL-1β, IL-6, IL-8, IL-10, IL-31, tumor necrosis factor (TNF)-α, and transforming growth factor (TGF)-β in sweat (Didierjean et al., 1990; Marques-Deak et al., 2006; Sato & Sato, 1994). It is possible that sweat cytokines are at least partially derived from circulating stores, as some studies have found no statistical differences between sweat and plasma concentrations at rest (Marques-Deak et al., 2006). However, it seems that cytokines may also originate from the sweat gland itself since cytokines are innately expressed in eccrine glands. For example, the eccrine gland has been shown to express immunohistochemical activity of various cytokines (IL-1α, IL-1β, IL-6, IL-8, IL-31, TGF-β, and TNF-α) as well mRNA encoding from in situ hybridization studies ((IL-1α, IL-1β, IL-8, TNF-α) (Baker & Wolfe, 2020). Furthermore, elevated sweat cytokine concentrations during exercise and thermal stress (Didierjean et al., 1990; Sato & Sato, 1994) would indicate stress-induced cytokine secretion rather than origination from the blood. Nonetheless, more research is needed to elucidate potential mechanisms of cytokine transport from the plasma as well as production by the eccrine glands.
Evidence as a Biomarker. Like salivary cortisol, there is interest in using sweat cortisol as a non-invasive biomarker for monitoring stress and circadian-related disorders to aid in the prevention of disease or acceleration of recovery. Sweat cortisol follows a diurnal pattern (Kim et al., 2020) and concentrations have been shown to be similar to salivary cortisol concentrations (Russell et al., 2014; Torrente-Rodriguez et al., 2020). However, only one study has compared cortisol concentrations in sweat versus blood. In a pilot study, a wearable device was used to measure sweat cortisol during exercise and a cold pressor test designed to induce physical stress. When all data were pooled (8 subjects, 4 data points each) there was a significant correlation between sweat and serum (r=0.87) and sweat and saliva cortisol concentrations (r=0.78) (Torrente-Rodriguez et al., 2020). More research is needed, however, to determine the utility of sweat cortisol as a biomarker in sport applications.
There is interest in the potential of sweat cytokines to serve as non-invasive biomarkers to monitor immune system function or local skin inflammation. Cytokine concentrations of samples collected during passive (insensible) sweating have been shown to be higher in patients with major depressive disorder (Cizza et al., 2008) and influenza/cold symptoms (Jagannath et al., 2021) versus healthy controls. In addition, two studies from the same laboratory (Cizza et al., 2008; Marques-Deak et al., 2006) have reported significant correlations between cytokine concentrations (IL-1α, IL-1β, TNF-α, IL-6, IL-8, TGF-β) measured in insensible sweat (collected over a 24-h period) and plasma samples (collected toward the end of the sweat collection period). However, more work is needed to corroborate these findings and determine the utility of sweat cytokines as biomarkers in athlete health and wellness.
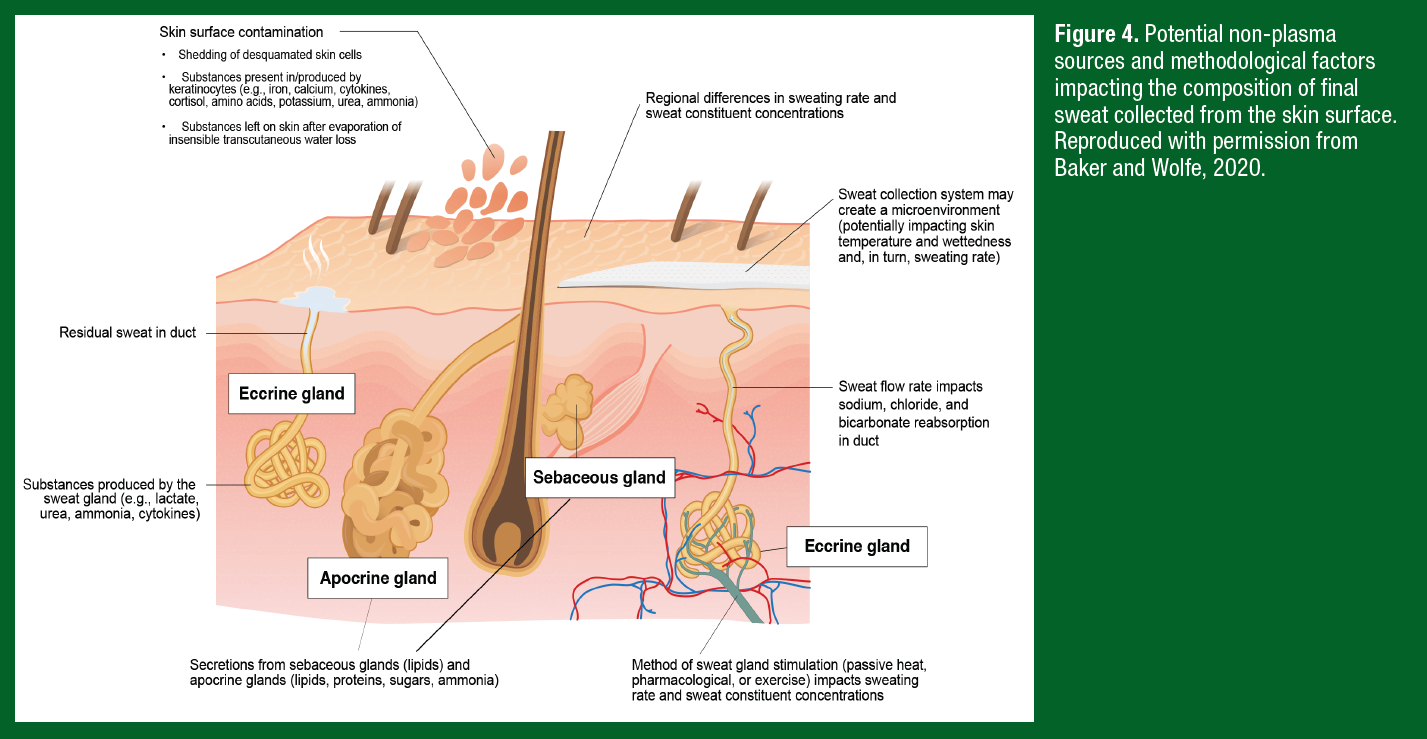
CHALLENGES
Because extracellular fluid is the precursor fluid for primary sweat, it follows that many components of the blood plasma are also found in final sweat. However, there are several other factors dictating solute concentrations of final sweat collected from the skin surface, which generally fall into one of three categories: non-plasma sources, methodological, and intra/interindividual factors. As discussed previously, some substances do not originate from precursor sweat (i.e., extracellular fluid/plasma), but instead enter the sweat gland because of production by the eccrine gland or appear in final sweat on the skin surface via contact with keratinocytes. These non-plasma sources of sweat content are illustrated in Figure 4.
The methodological factors that influence sweat flow rate and composition include the mode of sweat stimulation, type of sweat collection system, and region of sweat collection (Figure 4). While many wearable sensor studies collect sweat during active sweating (i.e., exercise), as discussed above there is also interest in sweat biomarker monitoring during rest/recovery. Thus, some wearables are designed for collection of microliter volumes of fluid during passive sweating; either chronic insensible losses or acute stimulation via an integrated cholinergic agent (e.g., carbachol, pilocarpine, etc.). However, sweating rates during exercise can be 2-3 times higher than that of passive sweating. The method of sweat stimulation can also impact sweat electrolyte, trace mineral, and metabolite concentrations. For example, sweat metabolite concentrations are typically higher with active versus passive sweating, but interpretation of these results is difficult since the data are confounded by differences in sweating rates and other study limitations (for review see Baker & Wolfe, 2020).
Sensor studies have employed a wide range of approaches to collect sweat, including adhesive patches, temporary tattoos, wrist bands, off-body fingertip sensors, nose pads on glasses, and textiles. It is unknown how the device/material itself could influence sweat flow rate and sweat composition by creating a microclimate localized to the covered skin surface. Studies suggest that the effect of standard collection techniques on skin microclimate varies depending upon the duration of application and ambient temperature. Skin temperature is more likely to be elevated (compared with noncovered skin) when absorbent patches are worn for longer durations (e.g., 60 min) or in temperate environments (Klous, Folkerts, et al., 2021). Higher skin temperature would be expected to result in elevated sweating rate. By contrast, elevated skin wettedness from wearing an occlusive covering for a prolonged period of time may lead to hidromeiosis, a condition that causes a gradual decline in sweating rate (Collins & Weiner, 1962). Finally, it is well known that there are significant regional differences in sweat distribution and composition. Studies have reported ~3-6 fold differences in sweating rate and ~2-4 fold differences in electrolyte, trace mineral, lactate, amino acid, and bicarbonate concentrations across the body (Baker & Wolfe, 2020).
Interindividual factors, such as heat acclimation status, diet, disease, medication, tattoos, sex, age/maturation, among others, may have further effects on sweat solute concentrations that could confound interpretation of results depending upon the biomarker and application of interest. However, the impact of these factors is largely unknown for most constituents with perhaps the exception of Na+ and Cl-, as discussed above. It is also important to note that changes in exercise intensity or environmental conditions that elicit changes in sweating rate are likely to impact final sweat composition (particularly Na+, Cl-, lactate, urea, and bicarbonate) (Baker & Wolfe, 2020). Finally, there is a high degree of interindividual variability for many biomarkers discussed, even when measured in the blood, (e.g., cortisol). Thus, baseline measures and considerations for time lag from circulation to sweat may also need to be considered for interpretation of results.
CONCLUSION
Despite recent technological advances in wearable devices and considerable interest in using sweat as a non-invasive alternative to blood, the application of sweat diagnostics in sports science has been limited to date. This is at least partly because noise in the measurement of sweat composition and its independency from blood concentrations can make it challenging to know whether to interpret changes in sweat constituent concentration as physiologically meaningful. The utility of sweat as a biomarker may depend upon the precision required for the desired application – e.g., detecting large differences in nutrient status via sweat may be more feasible than small, real-time changes in blood metabolite concentrations. Furthermore, there are still key technical challenges in the field of wearable sweat sensing such as handling a wide range of flow conditions, analytical sensitivity, miniaturization, and energy/memory requirements for continuous operation. Future studies should address important gaps in the literature and account for key methodological factors to elucidate the potential utility of sweat as a proxy for blood and/or as a biomarker for physiological/nutritional status.
PRACTICAL APPLICATIONS
- Measuring sweat electrolyte concentrations is useful for quantifying NaCl losses as a consequence of sweating during exercise, but not as a biomarker for real-time assessment of hydration status (i.e., fluid balance) per se.
- Trace mineral and vitamin concentrations in sweat generally increase after oral administration, but there are few well-controlled studies comparing sweat vs. blood.
- There is little to no evidence of a correlation between sweat and blood metabolites, nitrogenous wastes or stress and immune markers, especially in healthy individuals at rest or during exercise.
- Some preliminary data collected with novel wearable devices have reported significant correlations between sweat and blood for glucose and lactate after incorporating algorithms to calibrate for individual differences and account for blood-sweat time lags. However, well-designed clinical trials are needed to corroborate these findings.
- Concentrations of sweat constituents are at least partially independent of blood concentrations because of ductal reabsorption (Na+, Cl-, bicarbonate) or production by the eccrine glands (e.g., lactate, urea, ammonia, cytokines) or skin (e.g., urea, ammonia, amino acids, Ca2+, Fe2+).
- In future research it is important to use sweat stimulation methods that are fit for the intended question and application of the data (e.g., local or whole body, pharmacological or thermal, resting 24-h vs. acute exercise).
Authors are employed by the Gatorade Sports Science Institute, a division of PepsiCo R&D. The views expressed are those of the authors and do not necessarily reflect the position or policy of PepsiCo, Inc.
REFERENCES
Alvear-Ordenes, I., Garcia-Lopez, D., De Paz, J. A., & Gonzalez-Gallego, J. (2005). Sweat lactate, ammonia, and urea in rugby players. Int J Sports Med, 26(8), 632-637.
Ament, W., Huizenga, J. R., Mook, G. A., Gips, C. H., & Verkerke, G. J. (1997). Lactate and ammonia concentration in blood and sweat during incremental cycle ergometer exercise. Int J Sports Med, 18(1), 35-39.
Armstrong, L. E., Hubbard, R. W., Szlyk, P. C., Matthew, W. T., & Sils, I. V. (1985). Voluntary dehydration and electrolyte losses during prolonged exercise in the heat. Aviat Space Environ Med, 56(8), 765-770.
Baker, L. B., De Chavez, P. J. D., Ungaro, C. T., Sopena, B. C., Nuccio, R. P., Reimel, A. J., & Barnes, K. A. (2019). Exercise intensity effects on total sweat electrolyte losses and regional vs. whole-body sweat [Na(+)], [Cl(-)], and [K(+)]. Eur J Appl Physiol, 119(2), 361-375.
Baker, L. B., & Wolfe, A. S. (2020). Physiological mechanisms determining eccrine sweat composition. Eur J Appl Physiol, 120(4), 719-752.
Barnes, K. A., Anderson, M. L., Stofan, J. R., Dalrymple, K. J., Reimel, A. J., Roberts, T. J., Randell, R. K., Ungaro, C. T., & Baker, L. B. (2019). Normative data for sweating rate, sweat sodium concentration, and sweat sodium loss in athletes: An update and analysis by sport. J Sports Sci, 37(20), 2356-2366.
Boysen, T. C., Yanagawa, S., Sato, F., & Sato, K. (1984). A modified anaerobic method of sweat collection [Research Support, U.S. Gov't, P.H.S.]. J Appl Physiol, 56(5), 1302-1307.
Brown, M. B., Haack, K. K., Pollack, B. P., Millard-Stafford, M., & McCarty, N. A. (2011). Low abundance of sweat duct Cl- channel CFTR in both healthy and cystic fibrosis athletes with exceptionally salty sweat during exercise. Am J Physiol Regul Integr Comp Physiol, 300(3), R605-615.
Brusilow, S. W. (1967). Evidence for a non-plasma source of urea in sweat. Nature, 214(5087), 506.
Brusilow, S. W., & Gordes, E. H. (1968). Ammonia secretion in sweat. Am J Physiol, 214(3), 513-517.
Buono, M. J., Claros, R., Deboer, T., & Wong, J. (2008). Na+ secretion rate increases proportionally more than the Na+ reabsorption rate with increases in sweat rate. J Appl Physiol (1985), 105(4), 1044-1048.
Buono, M. J., Lee, N. V., & Miller, P. W. (2010). The relationship between exercise intensity and the sweat lactate excretion rate. J Physiol Sci, 60(2), 103-107.
Cage, G. W., & Dobson, R. L. (1965). Sodium Secretion and Reabsorption in the Human Eccrine Sweat Gland. J Clin Invest, 44, 1270-1276.
Choi, J. Y., Muallem, D., Kiselyov, K., Lee, M. G., Thomas, P. J., & Muallem, S. (2001). Aberrant CFTR-dependent HCO3- transport in mutations associated with cystic fibrosis. Nature, 410(6824), 94-97.
Cizza, G., Marques, A. H., Eskandari, F., Christie, I. C., Torvik, S., Silverman, M. N., Phillips, T. M., Sternberg, E. M., & Group, P. S. (2008). Elevated neuroimmune biomarkers in sweat patches and plasma of premenopausal women with major depressive disorder in remission: the POWER study. Biol Psychiatry, 64(10), 907-911.
Collins, K. J., & Weiner, J. S. (1962). Observations on arm-bag suppression of sweating and its relationship to thermal sweat-gland 'fatigue'. J Physiol, 161, 538-556.
Costill, D. L. (1977). Sweating: its composition and effects on body fluids. Ann N Y Acad Sci, 301, 160-174.
Cui, C. Y., & Schlessinger, D. (2015). Eccrine sweat gland development and sweat secretion. Exp Dermatol, 24(9), 644-650.
Czarnowski, D., Gorski, J., Jozwiuk, J., & Boron-Kaczmarska, A. (1992). Plasma ammonia is the principal source of ammonia in sweat. Eur J Appl Physiol Occup Physiol, 65(2), 135-137.
Di Sant'Agnese, P. A., Darling, R. C., Perera, G. A., & Shea, E. (1953). Abnormal electrolyte composition of sweat in cystic fibrosis of the pancreas; clinical significance and relationship to the disease. Pediatrics, 12(5), 549-563.
Didierjean, L., Gruaz, D., Frobert, Y., Grassi, J., Dayer, J. M., & Saurat, J. H. (1990). Biologically active interleukin 1 in human eccrine sweat: site-dependent variations in alpha/beta ratios and stress-induced increased excretion. Cytokine, 2(6), 438-446.
Dunstan, R. H., Sparkes, D. L., Dascombe, B. J., Macdonald, M. M., Evans, C. A., Stevens, C. J., Crompton, M. J., Gottfries, J., Franks, J., Murphy, G., Wood, R., & Roberts, T. K. (2016). Sweat Facilitated Amino Acid Losses in Male Athletes during Exercise at 32-34 degrees C. PLoS One, 11(12), e0167844.
Dziedzic, C. E., Ross, M. L., Slater, G. J., & Burke, L. M. (2014). Variability of Measurements of Sweat Sodium Using the Regional Absorbent Patch Method. Int J Sports Physiol Perform.
Gibinski, K., Zmudzinski, J. Z. J., Kumaszka, F., Giec, L., & Waclawczyk, J. (1974). Calcium transit to thermal sweat. Acta Biol Med Ger, 32(2-3), 199-204.
Gitlitz, P. H., Sunderman, F. W., Jr., & Hohnadel, D. C. (1974). Ion-exchange chromatography of amino acids in sweat collected from healthy subjects during sauna bathing. Clin Chem, 20(10), 1305-1312.
Green, J. M., Bishop, P. A., Muir, I. H., McLester, J. R., Jr., & Heath, H. E. (2000). Effects of high and low blood lactate concentrations on sweat lactate response. Int J Sports Med, 21(8), 556-560.
Huang, C. T., Chen, M. L., Huang, L. L., & Mao, I. F. (2002). Uric acid and urea in human sweat. Chin J Physiol, 45(3), 109-115.
Jagannath, B., Lin, K. C., Pali, M., Sankhala, D., Muthukumar, S., & Prasad, S. (2021). Temporal profiling of cytokines in passively expressed sweat for detection of infection using wearable device. Bioeng Transl Med, 6(3), e10220.
Jenkins, M. E., Rivarola, M. A., Brusilow, S. W., & Migeon, C. J. (1969). Excretion of 4-14C-cortisol and 1,2-3H-D-aldosterone in human thermal sweat. J Clin Endocrinol Metab, 29(8), 1102-1106.
Kaiser, D., Songo-Williams, R., & Drack, E. (1974). Hydrogen ion and electrolyte excretion of the single human sweat gland. Pflugers Arch, 349(1), 63-72.
Kim, J., Wu, Y., Luan, H., Yang, D. S., Cho, D., Kwak, S. S., Liu, S., Ryu, H., Ghaffari, R., & Rogers, J. A. (2022). A Skin-Interfaced, Miniaturized Microfluidic Analysis and Delivery System for Colorimetric Measurements of Nutrients in Sweat and Supply of Vitamins Through the Skin. Adv Sci (Weinh), 9(2), e2103331.
Kim, S., Lee, B., Reeder, J. T., Seo, S. H., Lee, S. U., Hourlier-Fargette, A., Shin, J., Sekine, Y., Jeong, H., Oh, Y. S., Aranyosi, A. J., Lee, S. P., Model, J. B., Lee, G., Seo, M. H., Kwak, S. S., Jo, S., Park, G., Han, S., . . . Rogers, J. A. (2020). Soft, skin-interfaced microfluidic systems with integrated immunoassays, fluorometric sensors, and impedance measurement capabilities. Proc Natl Acad Sci U S A, 117(45), 27906-27915.
Klous, L., de Ruiter, C. J., Scherrer, S., Gerrett, N., & Daanen, H. A. M. (2021). The (in)dependency of blood and sweat sodium, chloride, potassium, ammonia, lactate and glucose concentrations during submaximal exercise. Eur J Appl Physiol, 121(3), 803-816.
Klous, L., Folkerts, M., Daanen, H., & Gerrett, N. (2021). The effect of short and continuous absorbent patch application on local skin temperature underneath. Physiol Meas, 42(4).
Komives, G. K., Robinson, S., & Roberts, J. T. (1966). Urea transfer across the sweat glands. J Appl Physiol, 21(6), 1681-1684.
Mark, H., & Harding, C. R. (2013). Amino acid composition, including key derivatives of eccrine sweat: potential biomarkers of certain atopic skin conditions. Int J Cosmet Sci, 35(2), 163-168.
Marques-Deak, A., Cizza, G., Eskandari, F., Torvik, S., Christie, I. C., Sternberg, E. M., Phillips, T. M., & Premenopausal, O. W. A. D. S. G. (2006). Measurement of cytokines in sweat patches and plasma in healthy women: validation in a controlled study. J Immunol Methods, 315(1-2), 99-109.
McCubbin, A. J., Lopez, M. B., Cox, G. R., Caldwell Odgers, J. N., & Costa, R. J. S. (2019). Impact of 3-day high and low dietary sodium intake on sodium status in response to exertional-heat stress: a double-blind randomized control trial. Eur J Appl Physiol, 119(9), 2105-2118.
Mickelsen, O., & Keys, A. (1943). The composition of sweat, with special reference to the vitamins. J Biol Chem, 149, 479-490.
Milne, D. B., Canfield, W. K., Mahalko, J. R., & Sandstead, H. H. (1983). Effect of dietary zinc on whole body surface loss of zinc: impact on estimation of zinc retention by balance method. Am J Clin Nutr, 38(2), 181-186.
Montagna, W., & Parakkal, P. F. (1974a). Apocrine Glands. In W. Montagna & P. F. Parakkal (Eds.), The Structure and Function of Skin (Third ed., pp. 332-365). Academic Press, Inc.
Montagna, W., & Parakkal, P. F. (1974b). Sebaceous Glands. In W. Montagna & P. F. Parakkal (Eds.), The Structure and Function of Skin (Third ed., pp. 280-331). Academic Press, Inc.
Morgan, R. M., Patterson, M. J., & Nimmo, M. A. (2004). Acute effects of dehydration on sweat composition in men during prolonged exercise in the heat. Acta Physiol Scand, 182(1), 37-43.
Nose, K., Mizuno, T., Yamane, N., Kondo, T., Ohtani, H., Araki, S., & Tsuda, T. (2005). Identification of ammonia in gas emanated from human skin and its correlation with that in blood. Anal Sci, 21(12), 1471-1474.
Patterson, M. J., Galloway, S. D., & Nimmo, M. A. (2002). Effect of induced metabolic alkalosis on sweat composition in men. Acta Physiol Scand, 174(1), 41-46.
Prasad, A. S., Schulert, A. R., Sandstead, H. H., Miale, A., Jr., & Farid, Z. (1963). Zinc, iron, and nitrogen content of sweat in normal and deficient subjects. J Lab Clin Med, 62, 84-89.
Quinton, P. M. (1999). Physiological basis of cystic fibrosis: a historical perspective. Physiol Rev, 79(1 Suppl), S3-S22.
Quinton, P. M., & Reddy, M. M. (1989). Cl- conductance and acid secretion in the human sweat duct. Ann N Y Acad Sci, 574, 438-446.
Russell, E., Koren, G., Rieder, M., & Van Uum, S. H. (2014). The detection of cortisol in human sweat: implications for measurement of cortisol in hair. Ther Drug Monit, 36(1), 30-34.
Sato, K. (1977). The physiology, pharmacology, and biochemistry of the eccrine sweat gland. Rev Physiol Biochem Pharmacol, 79, 51-131.
Sato, K., & Dobson, R. L. (1970). The effect of intracutaneous d-aldosterone and hydrocortisone on human eccrine sweat gland function. J Invest Dermatol, 54(6), 450-462.
Sato, K., & Dobson, R. L. (1973). Glucose metabolism of the isolated eccrine sweat gland. II. The relation between glucose metabolism and sodium transport. J Clin Invest, 52(9), 2166-2174.
Sato, K., Kang, W. H., Saga, K., & Sato, K. T. (1989). Biology of sweat glands and their disorders. II. Disorders of sweat gland function. J Am Acad Dermatol, 20(5 Pt 1), 713-726.
Sato, K., & Sato, F. (1994). Interleukin-1 alpha in human sweat is functionally active and derived from the eccrine sweat gland. Am J Physiol, 266(3 Pt 2), R950-959.
Shibasaki, M., & Crandall, C. G. (2010). Mechanisms and controllers of eccrine sweating in humans. Front Biosci (Schol Ed), 2, 685-696.
Strauss, J. S., Downing, D. T., & Ebling, F. J. (1983). Sebaceous Glands. In L. A. Goldsmith (Ed.), Biochemistry and Physiology of the Skin (Vol. 1, pp. 569-595). Oxford University Press, Inc.
Torrente-Rodriguez, R. M., Tu, J., Yang, Y., Min, J., Wang, M., Song, Y., Yu, Y., Xu, C., Ye, C., IsHak, W. W., & Gao, W. (2020). Investigation of cortisol dynamics in human sweat using a graphene-based wireless mHealth system. Matter, 2(4), 921-937. https://doi.org/10.1016/j.matt.2020.01.021
Vellar, O. D. (1968). Studies on sweat losses of nutrients. I. Iron content of whole body sweat and its association with other sweat constituents, serum iron levels, hematological indices, body surface area, and sweat rate. Scand J Clin Lab Invest, 21(2), 157-167.
Walsh, R. M., Noakes, T. D., Hawley, J. A., & Dennis, S. C. (1994). Impaired high-intensity cycling performance time at low levels of dehydration. Int J Sports Med, 15(7), 392-398.
Xie, L., Jin, L., Feng, J., & Lv, J. (2017). The Expression of AQP5 and UTs in the Sweat Glands of Uremic Patients. Biomed Res Int, 2017, 8629783.
Yang, Y., Song, Y., Bo, X., Min, J., Pak, O. S., Zhu, L., Wang, M., Tu, J., Kogan, A., Zhang, H., Hsiai, T. K., Li, Z., & Gao, W. (2020). A laser-engraved wearable sensor for sensitive detection of uric acid and tyrosine in sweat. Nat Biotechnol, 38(2), 217-224.
Yoshida, T., Shin-ya, H., Nakai, S., Yorimoto, A., Morimoto, T., Suyama, T., & Sakurai, M. (2006). Genomic and non-genomic effects of aldosterone on the individual variation of the sweat Na+ concentration during exercise in trained athletes. Eur J Appl Physiol, 98(5), 466-471.
Zhao, J., Nyein, H. Y. Y., Hou, L., Lin, Y., Bariya, M., Ahn, C. H., Ji, W., Fan, Z., & Javey, A. (2021). A Wearable Nutrition Tracker. Adv Mater, 33(1), e2006444.