KEY POINTS
- The integration of accurate, real-time wearable sensors represents a unique opportunity to protect the health of athletes during training and competition, as demonstrated in pilot implementations during the Tokyo 2020 Olympics and 2022 Adidas Road to Records.
- These advancements represent a significant progression in the use of wearable technologies in exercise physiology, in which initial endeavours date back to the early 1900s.
- The monitoring of meteorological conditions, prioritizing wet bulb globe temperature (WBGT) in situ at the athletes’ specific location during competition, offers precise information to protect athletes from exertional heat illnesses.
- Despite the undoubted benefits of real-time wearables, there are well-founded concerns relating to insufficient validity testing, data privacy and information overload.
- The rise of biometrics has relevance for broadcasting, as well as sports performance professionals.
- Remote monitoring technology frameworks developed in sport have the potential to be applied in public health settings and used by first responders and the military.
INTRODUCTION HISTORICAL CONTEXT
Wearable technology is experiencing exponential growth in the realms of sport, physical activity and health. In sports, global positioning system (GPS) devices, smartwatches and biosensors are helping to guide the way athletes, sports prosumers and longevity lifestylers eat, sleep, train and perform. For the wider population, activity trackers, stress monitors and virtual reality wearables help to refine our daily routines and motivate us to lead more physically active lifestyles. Whilst in health, remote sensor technologies represent a new frontier for preventive healthcare and real-time monitoring of people with illnesses such as diabetes and those recovering from surgery, accidents or injury. Smartwatches and smart bands can continuously monitor vital signs such as heart rate (HR), blood pressure (BP) and blood oxygen levels, supporting individualised Telemedicine, thereby increasing healthcare access. Yet, despite the increased attention that such microelectronics technology has received in the past decade and its apparent newness, the notion of ‘wearable’ technology is not a new phenomenon in the field of exercise physiology.
The inception of the Douglas Bag in the early 20th century enabled pioneers of our field to conduct experiments outside of laboratories. Photographic evidence demonstrated attempts to collect respiratory gases during both outdoor boxing and running (Hill et al., 1924). In the decades that followed, wearable technologies of the time contributed to step-changes in our understanding of physiology. Scientists began to use portable technologies to collect measurements in novel locations or with previously inaccessible populations. For example, Pugh (1958) used semi-portable Douglas bags during an early Everest expedition. In 1978, the company Polar (2023) filed its first wireless HR monitor patent. By the 1990s and early 2000s, researchers were taking early iterations of wearable oxygen analysers to the highlands of Ethiopia and Kenya, as awareness of the East Africa running phenomenon began (Longman 2016). Fast forward into the 21st century and wearable technologies appear commonplace for both elite and amateur sportspeople and continue to rewrite our understanding of human physiology. Data collected from ingestible temperature pills has quashed conventional wisdom and the Critical Core Temperature theory (Racinais et al., 2019). Whilst in team sports, players now routinely train and compete with GPS/accelerometer devices to monitor locomotor activity, enabling granular assessment of both training and competition demands (Aughey, 2011).
We postulate that the next major development within wearables and exercise physiology is the transition away from singular sensors and the integration of multiple sensor technologies into a single suite, with live data transmission. As set out in the International Olympic Committee (IOC) consensus on sporting events in the heat (Racinais et al., 2023), the use of technology to monitor athletes’ physiological and biomechanical performance indicators in real time during competition is needed for the prevention of injury and illness. For example, such technology can enable medical teams to make prompt and crucial decisions around athletes continuing to compete or withdrawing from an event.
To characterize these recent developments, in this Sports Science Exchange (SSE) article, we describe and review two high profile sporting events where innovations in wearable technologies were trialled: the Tokyo 2020 Summer Olympic Games (Tokyo 2020, Japan), and the 2022 Adidas Road to Records event (Germany). These two major sporting events demonstrated academic and industry partners coming together to implement real-time wearable solutions to protect the health of athletes competing in hot and humid environments and to better understand how these metrics can be used moving forward.
TOKYO 2020
This project followed discussions by members of the Adverse Weather Impact Expert Working Group of the IOC, which was created to proactively protect the health of athletes during Tokyo 2020, given the anticipated extreme environmental conditions (Hosokawa et al., 2021; Muniz-Pardos et al., 2021a). The IOC Group initiated this project to build on the success of data collection at the Doha 2019 World Athletics Championships, where core body temperature (TCORE) and the impact of different cooling strategies were assessed during the championships using live-transmitting technology and thermal cameras (Racinais et al., 2022). The current approach developed this, combining TCORE and skin temperature responses that may be associated with collapse and/or withdrawal from competition with biomechanical parameters that can identify disturbances in gait and body sway. Collectively, this data may help medical staff in the early identification of possible aggravated hyperthermia situations (Buller et al., 2022). This approach was enabled by the development of a smartwatch application and ecosystem designed to collect, process and transmit physiological, biomechanical, bioenergetic and environmental data using cloudbased services (Muniz-Pardos et al., 2018). Athlete support teams and relevant stakeholders can view the data in real time, anywhere with internet or mobile access (Duking et al., 2018; Muniz-Pardos et al., 2019). This technology has the potential to help in the management of athletes during a medical emergency to instantly orient the diagnosis and accelerate delivery of care.
TOKYO 2020 METHODS
A cross-sectional observational and descriptive approach was used. The methods previously described in detail (Muniz-Pardos et al., 2018; 2021b) enabled biomechanical, physiological and bioenergetic monitoring, across identified Olympic sports at risk of exertional heat stroke. In the weeks prior to Tokyo 2020, athletes were invited to participate via their National Olympic Committee team physicians. No athletes/coaches were recruited during the Games, in line with the agreed IOC approval process. Unfortunately, various Covid-19 restrictions prevented more widespread monitoring at the Games (Pigozzi et al., 2021), not least due to limited accreditation of the research group (n=2 researchers). Therefore, whilst some discussions and feasibility work were conducted across windsurfing, sailing, equestrian and open water swimming, for this article we focus on data collection (from two athletes) participating in specific Olympic events (10,000 m, marathon and 20 km race walk). Both athletes agreed to have TCORE measured as the primary outcome, achieved by swallowing a temperature pill 6 hours prior to their event. There were a range of other optional metrics, as detailed below. Athletes could personalize the smartwatch data display, as well as select real-time transmission or offline recording for their data. Real-time monitoring was achieved by a small wrist worn ‘gateway’ bracelet that, alongside a smartwatch and bespoke ‘Sub2’ application, transmitted data via the cellular network to the research team (Figure 1). Cellular connectivity in Japan was achieved using e-SIMs and the smartwatch set in roaming (Guppy et al., 2023). In addition to TCORE, athletes consented to at least one of the following measurements during training and/or competition.
Heart Rate.
Telemetric chest strap or via inbuilt smartwatch. Chest strap monitors were encouraged over the wrist sensor for greater accuracy during exercise (Fudge et al., 2007).
Skin Temperature.
Temperature pills were adapted by the manufacturer to enable skin temperature measurement, by “flattening” the pill electronics to ensure the thermistor always contacted the skin. The sensor was affixed using a standard HR strap.
Stride and Foot Mechanics Sensor.
A foot-worn inertial sensor was placed on the laces of each shoe. The sensor measures contact time (s) and strike angle (deg) of each foot, cadence (steps/min), and foot mechanics variability. The above-mentioned variables were chosen to explore the variability of ground contact time as a potential indicator of a heat stroke or injury, as well as the change of contact time during the race as a marker of fatigue. Given that cadence and footstrike angles reflect runners’ commitment, performance strategies (i.e., acceleration, deceleration, steady-state pace, etc.) and techniques (i.e., forefoot strikes, midfoot strikes, etc.) respectively, this data and the variability within, may indicate fatigue, injury or heat stroke (Girard et al., 2016).
ENVIRONMENTAL CONDITIONS
For monitoring ambient conditions, Wet Bulb Globe Temperature (WBGT) remains the ubiquitous approach. Most portable devices do not measure all components of WBGT, for example, no aspirated ambient air temperature. However, the ‘Kestrel’ portable WBGT monitor is considered a reasonable compromise and acceptable for use in field monitoring studies (Cooper et al., 2017; Falconer Hall et al., 2020). Nevertheless, the environmental conditions that an athlete experiences can vary considerably across large competition locations (e.g. 42 km marathon route). Therefore, duplicate approaches for assessing environmental conditions across venues were explored. Ambient temperature was monitored and recorded using Kestrel devices at all Olympic venues where the project took place. Kestrel devices were linked by Bluetooth to the real-time system via a bespoke cellular wireless transmitter. The application provided live data air and land surface temperatures, and relative humidity (Buller et al., 2022). Using static weather stations to gauge air temperature and relative humidity may not fully account for the spatial variations of these metrics, given the network’s limited coverage. A unique development briefly described elsewhere (Muniz-Pardos et al., 2018) enables tracking of the actual heat experience of the individual (i.e., the SCOUTS model) (Tonekaboni et al., 2018). The SCOUTS model was designed to minimize heat stress in individuals and urban communities by using “Mobile Crowdsensing”, which allows the model to gather data at finer spatial-temporal granularities compared to traditional methods. Furthermore, a complimentary innovation involves downscaling satellite weather forecast data at the athlete’s location using advanced machine learning algorithms. This is especially pertinent in regions where weather station networks are absent. This approach can be applied to any global location and provide ambient conditions for each athlete. Importantly, there are endless possibilities to scale up such monitoring, for example to include more parameters such as the forecast of upcoming ambient conditions, UV index and air quality indices. The technological solution we applied (www.extrema-global.com) integrates real-time data transmission including ambient conditions from downscaled modelled data via an Application Programming Interface connection in pre-designated areas (e.g. Sapporo, where marathon and race-walking events occurred). See Table 1 for ambient conditions during three Olympic events at Tokyo 2020.
TOKYO 2020 RESULTS
10,000 m Men’s Olympic Final (Tokyo, Japan).
A 10,000 m Olympic finalist volunteered to use the technology during the race, but opted not to wear the chest HR monitor, skin temperature sensor or the foot sensors. Therefore, a proprietary “physilog” watch-based running algorithm was used to collect spatial-temporal variables including ground contact time, cadence and running vertical oscillation, via a running biomechanics database integrated into the watch. This negated the need for foot sensors. The watch HR monitor revealed a high average HR (186 bpm) and a very high HRMAX (200 bpm) that may reflect a measurement artifact from the wrist-based sensor (Table 1). Average TCORE was high (39.5°C) with a maximum of 40.2°C reflecting the high intensity effort and characteristic of a 10,000 m final run during hot and humid conditions (Figure 2).
Men’s Marathon Olympic Final (Sapporo, Japan).
The same athlete volunteered to use the same metrics (chest strap HR, TCORE and ambient conditions) during the Olympic marathon, held in Sapporo 8 days later. Note the lower average HR (162 bpm) and lower HRMAX (190 bpm) achieved by this athlete during the marathon, run during similar ambient conditions as those experienced during the 10,000 m final a few days earlier (Table 1). This, and different bioenergetic demands of the events, may also explain the approximately 1.4°C lower average and maximum TCORE in this athlete during the marathon versus the 10,000 m final (Table 1).
Men’s 20 km Race Walk Olympic Final (Sapporo, Japan).
The second athlete was a race walker who volunteered to use the same metrics during the Olympic final (Table 1). Note the substantially higher average (178 vs. 168 bpm) and maximum (211 vs. 178 bpm) HR using the smartwatch device versus HR strap, respectively. This difference of ~10 bpm for average HR and ~33 bpm for HRMAX, most likely reflects measurement error from the smartwatch sensor. This athlete used a two-temperature pill strategy having taken one temperature pill in the morning and the other in the early afternoon before the (late) afternoon race (16:30 on August 5th, 2021). The similar high average (39.2 vs 39.2°C) and maximum (39.6 vs 39.8°C) TCORE measurements between pills, suggests both had passed the stomach and were unaffected by fluid ingestion. The high TCORE was comparable to the 10,000 m data and substantially higher than the marathon (+0.6- 1.0°C). This is likely a reflection of the hotter conditions during the race walk (WBGT 7-9°C higher during the race walk vs. 10,000 m and marathon; Table 1).
TOKYO 2020 DISCUSSION
Despite challenges presented by Covid-19 restrictions, we demonstrated that the integrative solution described here and elsewhere (Duking et al., 2018; Muniz-Pardos et al., 2018) can monitor health, performance and environmental data during the largest of sporting events. During the specific competitions, data were viewed on a live dashboard (Figure 2) by the research team in a remote location, demonstrating the ability to provide instantaneous feedback to stakeholders such as the athlete/ coach/scientist/doctor/broadcasters. This successful implementation demonstrates a clear pathway to routine monitoring during competitions that occur in extreme weather as is being explored by the IOC (Racinais et al., 2023).
The need for individualized, multi-sensor monitoring approaches is clearly demonstrated by the data collected during the 10,000 m final. Here, the athlete displayed a peak TCORE of 40.2°C. Such extreme hyperthermic responses would have been considered highly dangerous in the recent past (Nybo & Gonzalez-Alonso, 2015) and the athlete would not be permitted to continue during many laboratory assessments. However, with the advent of telemetry pills, the exceptional tolerance of, and large inter-individual variation between elite athletes, is becoming better understood (Racinais et al., 2019). Therefore, for live or predictive monitoring of heat illness, combinations of TCORE, skin temperature, HR and gait patterning are likely required to cater for individual responses, whilst protecting health.
Following Tokyo 2020, and as the Covid-19 restrictions were relaxed, this technology infrastructure was developed to serve as a “hub” to aggregate a larger range of data feeds. The overarching aims remained the same; to protect the health of athletes, help characterize and understand performance at an individual level, to learn how these applications can be used for other applications, such as healthcare in the community, protecting first responders/military personnel and, to enhance the broadcast of sporting events by relaying interesting performance biometrics to spectators.
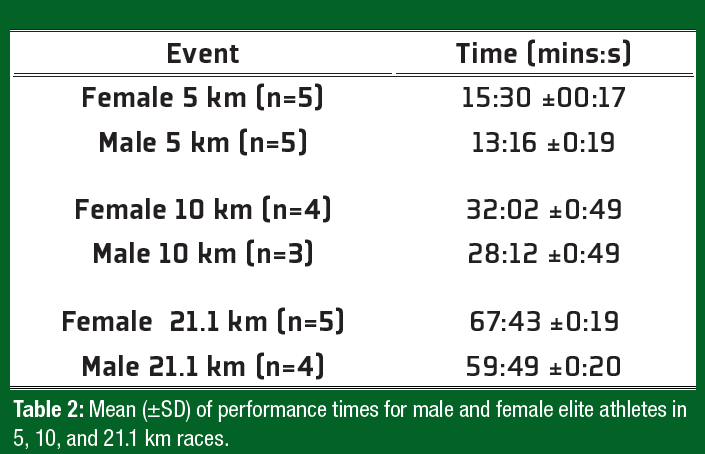
ADIDAS ROAD TO RECORDS
The Adidas Road To Records is a day of elite racing organized by Adidas, the sportswear manufacturer, held at Adidas HQ (Herzogenaurach, Bavaria) with the express aim of breaking records in the 5 km, 10 km and half marathon. Our team was invited to provide real-time monitoring of the performance of elite athletes in these events.
A major area of recent development in wearable technology is in measuring individual running biomechanics (Falbriard et al., 2018; Moran et al., 2015; Muniz-Pardos et al., 2019) in both athletic (Bushnell & Hunter, 2007) and clinical populations (Mariani et al., 2013), with the development of algorithms and computational power. The understanding and measurement of foot mechanics is crucial in endurance athletes, as optimizing factors such as foot strike patterns (Barnes & Kilding, 2015), lower ground reaction forces (Clark et al., 2017), shorter ground contact times (Nummela et al., 2007), greater stride angles (Santos-Concejero et al., 2014), lower cadence and longer strides (Tartaruga et al., 2012), can lead to improved running kinematics, and consequently, a reduced energy cost of running. These relationships are evidenced by the recent surge in world record performances using advanced footwear technology (Langley & Langley, 2023).
Historically, the assessment of running biomechanics is performed in the laboratory with intrinsic limitations, such as bouncy treadmills altering the biomechanical and bioenergetic response of athletes (Gidley et al., 2020). As such, monitoring of athlete’s performances during “real” events has been limited. However, portable sensors now support accurate assessments of running biomechanics in the field (Horsley et al., 2021). An interesting development within biomechanical metrics is the potential to detect gait perturbations, which may occur alongside the development of heat illness (Buller et al., 2022). Such patterns may have a role to play either individually or as part of a larger sensor suite to aid early detection of heat illness and protect athlete’s health. A major aim of this event was therefore to assess the feasibility of monitoring, in real time, multiple metrics of running mechanics of elite athletes taking part in the 5 km, 10 km and half marathon events.
ADIDAS ROAD TO RECORDS METHODS
Twenty-six elite athletes taking part in the Road to Records event agreed to wear multiple sensors during their events (5 km; n=10, 10 km; n=7 and 21.1 km; n=9). Data were transmitted live to the research team workstations and to the adidas live stream. Participants wore an inertial sensor in the shoelaces of one shoe as directed by Adidas. The sensor was equipped with a 9-axis IMU sensor (3-axis accelerometer, gyroscope and magnetometer) with a sampling frequency of up to 200 Hz and dynamic range of ± 16 g for high-intensity acceleration measurements. The sensor measured flight time, cadence and stride length for both the left and right leg, alongside a HR monitor. Satellitebased GPS data were used to visualize athletes’ position on the track and displayed on the live broadcast. These data were collated utilizing the same ecosystem previously detailed.
Due to the explorative nature of these data as well as the relatively small sample of elite athletes, no statistical analysis was conducted on this dataset. Quantitative data are presented as individual responses. All data handling was conducted within R (Rstudio, PBC, Boston, MA) utilising the tidyverse packages (Wickham et al., 2019) to visualize data and remove outliers (defined as >1.96 standard deviations from calculated mean).
ADIDAS ROAD TO RECORDS RESULTS
The mean performances of athletes during the Road to Records events are shown in Table 2. Cadence, flight time and stride length data are summarized in Table 3. Further, individual responses can be found in Guppy et al. (2023). Briefly, female runners displayed slower cadence values (in both feet), during the 5, 10 and 21.1 km races, compared with males. Females also displayed longer flight times compared to males across the 5 and 10 km events. However, flight times were similar between sexes during the 21.1 km event. Females displayed a shorter stride length across the 5 and 21.1 km events. However, in the 10 km, females showed a shorter stride length in the right but not left foot compared to males.
ADIDAS ROAD TO RECORDS DISCUSSION
We deliberately do not report large quantities of data to avoid over interpreting outcomes from a small sample of athletes. Our reporting is intended to allow researchers, support staff and athletes to assess the utility of incorporating this methodical approach. Nevertheless, we observed some intriguing patterns in our biomechanical data, both across short to long endurance events and between sexes.
The technology infrastructure worked well during this event, transmitting information to the research team location. Data were also shared in a user-friendly format with the host broadcaster, enabling Adidas management, coaches and online viewers to track athletes and gain insights into biometric data as athletes attempted to break the course records. As shown in Figure 3, athletes’ individual data may be captured and viewed live or post-race, second by second, enabling detailed performance monitoring such as HR responses.
With these exciting developments come challenges. Despite the small sample size, eleven performance variables generated between 1000 (5 km) and 5000 (21 km) data points per athlete. Considerable technical infrastructure is required for the appropriate utilization of this large amount of data. Technical skills amongst athlete support teams, and any potential future users of these approaches, need to be considered to ensure that the data are not simply collected but also utilized. We advocate data science training for future practitioners working in elite sport to fully utilize this technology for performance analytics.
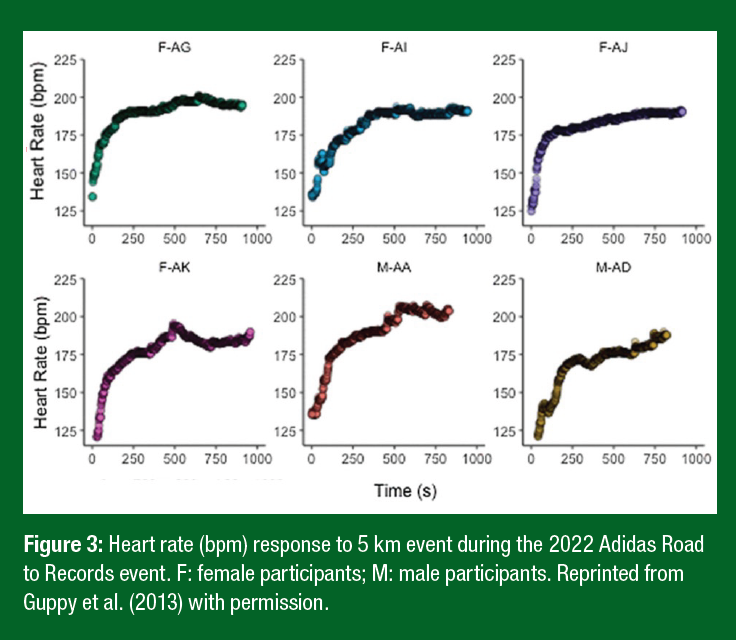
FUTURE RECOMMENDATIONS AND DIRECTIONS
As set out in the IOC consensus on sporting events in the heat (Racinais et al., 2023), the use of technology to monitor athlete’s physiological and biomechanical performance indicators in real time during a race is needed to develop effective injury and illness prevention strategies. These pilot studies show that athletes tolerate this technology, and a wide variety of variables can be monitored, allowing for new guardrails for athlete safety to be incorporated in the future.
Following Tokyo 2020, and after further pilot studies in Benasque (Aragon, Spain) and Singapore, the software application was redesigned to enable remote activation of data collection and to allow a greater number of athletes being tested at the same time (> 60). This avoids instances of athletes failing to activate, or in error deactivate, data streaming. This researcher- or support staff-controlled approach, is recommended to minimize interference by the athlete and supports an overarching philosophy of trying to achieve ‘invisible’ monitoring. The most pressing short-term application of these data are to provide useful information for supervising physicians who will be able to access live video feeds alongside the performance and biometrics of individual athletes. This helps to inform them of any clinical assessments around heat illnesses, that may be required. Such approaches have recently been discussed in the context of non-invasive TCORE measurement (Dolson et al., 2022) and are undoubtedly warranted given the ongoing rise in global temperatures.
SENSOR TECHNOLOGY AND INTEGRATION
A variety of (individual) wearable sensors worn on, close to, or even in the body that can monitor, analyse, transmit and/or receive data from other devices and/or cloud services are already in use or being developed (Ascaso & Huerva, 2016; Fuss et al., 2018; Lo Presti et al., 2017; Muniz-Pardos et al., 2018, 2019). At the individual sensor level, it is evident that trends of miniaturization will continue, as will increased user comfort, battery life and water resistance. This may include the wider use of smart garments and/or implanted sensors to address some of these issues.
The sensors presented in this paper are not novel when used in isolation. However, the integration within a real-time monitoring ecosystem is unique in competitive sport. It is evident that sensor suites of the future should be sensor agnostic, providing flexibility to display metrics of interest to various stakeholders and across different sports. For practitioners, it is not feasible to work with multiple applications for extracting or visualizing performance data. To facilitate this, consideration should be given to data integration within new sensors, such as Application Programming Interfaces (APIs) to minimize unnecessary data processing and maximize time for data interpretation.
A pertinent example of a relevant technology that can be integrated within the sensor suite we have trialled is the non-invasive, in situ, monitoring of sweating rate and sweat electrolyte losses. Through a skin-interfaced wearable microfluidic device, real-time personalized fluid-electrolyte intake recommendations could be provided (Baker et al., 2020). Another example are the ubiquitous microtechnologies already widely adopted in team sports. These devices incorporate Global Navigation Satellite Systems, accelerometery and gyroscopes enabling player’s locomotor activity to be recorded and reported live during training and competition (James et al., 2021a). For example, during match-play in rugby union (Hausler et al., 2016), as well as within a variety of other contexts, this technology has been in place for more than two decades and undergone extensive reliability and validity testing (Crang et al., 2021; Willmott et al., 2019). Similarly, local positioning systems are widely used by indoor sports, having shown to be valid systems to measure locomotion and positioning (Pino-Ortega et al., 2020). These provide team coaches and support staff with relevant locomotor metrics such as high-speed running distances, number of accelerations and decelerations and contacts for interpretation either alone, or in conjunction with tactical (e.g. team’s running outputs with/without ball possession) or contextual (e.g. opponent ranking or environmental conditions) (James et al., 2021b). Smart mouthguards containing built-in inertial measurement unit (IMU) sensors are another intriguing development for player safety, as concussion management becomes more sophisticated. Such devices help to monitor head kinematics and head impacts to estimate the degree of brain deformation, with validity and feasibility evidence emerging (Liu et al., 2020).
We believe one of the most powerful future applications of wearables will be the use of machine learning to integrate different data streams to develop algorithms that predict risk profiles for performance and health. A recent review demonstrates how multiple sensor technologies supports an algorithm to predict TCORE and to make decisions on athlete’s welfare (Dolson et al. 2022). A combination of biomechanics metrics (e.g. contact time, strike angle, acceleration/deceleration, foot mechanics and body sway (Ma et al., 2016)) may in time integrate with physiological data (e.g. TCORE, HR, sweat) and live environmental data, to provide a more robust interpretation than any singular sensor technology (Buller et al., 2022; Dolson et al., 2022). Such an approach would help address the considerable individual variability that is evident in heat tolerance across elite athletes (Racinais et al., 2019), reinforced by observations from the Tokyo 2020 10,000 m final (TCORE 40.2°C).
REGULATORY FRAMEWORK
As a result of the developments in wearables, sporting rules and regulations may need to be altered to facilitate their use. Some InternationalmFederations support using wearables, thereby encouraging companies to develop these tools which can support high-performance athletes. For example, Technical Rule 6.4.4 of the World Athletics Federations (2022) allows “Heart rate or speed distance monitors or stride sensors or similar devices carried or worn personally by athletes during an event, provided that such device cannot be used to communicate with any other person”. However, Union Cycliste Internationale (UCI) regulations on “Onboard Technology” (Chapter 3: Equipment) state “Devices which capture other physiological data, including any metabolic values such as but not limited to glucose or lactate are not authorized in competition”. Therefore, a re-evaluation of regulations will be required to support the use of wearables for key purposes of; (i) ensuring athlete safety, (ii) optimizing athletic performance through comprehensive monitoring of training and competition and (iii) maximizing the spectator experience.
BROADCASTING APPLICATIONS
Another key aspect of the developments we describe is the public engagement with these data and ability to gain insights into the performance of athletes. There is a drive amongst fans to better understand elite performance and the large amount of data generated in elite sport has started to make its way into the psyche of the spectator. A good example of this is the inclusion of strokes gained in golf (Broadie, 2012) which has influenced the strategic decisions being made by golfers of all levels. Sports such as squash and mountain biking have begun displaying live HR data during broadcasts, whilst strain meters provide an indication of the forces experienced by Formula 1 drivers. The evolution of the sporting event is going to include information availability to not only athletes, coaches and medical staff, but also to the spectator. This is well illustrated in the Tour de France’s partnership with Amaury Sports Organisation to connect fans to the heart of the action during both the Tour de France and the Tour de Femmes (Shei et al., 2022).
Making biometric data accessible to the public or other teams also brings new issues to be considered. Notably, performance/physiological data of athletes must be appropriately filtered and encrypted so that these cannot be used by other teams for competitive advantage. Additionally, sensors/technology used during competition, if providing a competitive advantage, must be accessible to all for fairness and equity during competition. There are also issues of ownership and use of intellectual property to be determined. These and other issues of ethics and fairness have been discussed in a recent editorial (Muniz- Pardos et al., 2021a).
MEASUREMENT ACCURACY
There are well-founded concerns regarding the lack of scientific peerreviewed papers concerning measurement accuracy of wearables across a number of scenarios coupled with exaggerated marketing claims by various manufacturers (Shei et al., 2022). Sensor technologies need to be established as safe, reliable and valid through high-quality independent research. This means that manufacturers and developers should substantiate marketing claims with independent scientific evidence so that global standards can be fully developed (Ash et al., 2021). Specific frameworks for these purposes within sports sciences are now emerging (Robertson et al., 2024). One challenge is that the algorithms that are used to determine these variables are the intellectual property of the companies developing the technologies. Establishing the robustness of the science in creating them will become a key aspect of this validation process. We therefore suggest that wearable devices that collect biological data for sport performance undergo rigorous evaluation ensuring that these devices have received a standard certification of veracity (Fury et al., 2021; Ponce-Bordon et al., 2022) or guiding reference (Ash et al., 2021) prior to acceptance of use in elite sporting competition. This quality control process and resulting “kitemark” of quality would undoubtedly be highly sought after for those individuals using them for general health monitoring.
INFORMATION OVERLOAD
The integration of multi-source data into a single dashboard presents considerable challenges around data interpretation and prioritization. To avoid ‘drowning in data’ it is recommended that users undertake data collection with clearly identified questions to answer. For example, whether the overarching aim is to support performance analysis of the event, interpret physiological responses (e.g. assess a training effect/ effect of intervention) or to support health monitoring. The development of the technology infrastructure we describe involved microelectronics engineers, data engineers, software engineers and telecommunications specialists, while sport scientists, coaches or medics may ultimately be the ones interpreting and acting upon data. It is evident therefore that future development of wearables will continue to require a multidisciplinary approach for effective implementation (Seckin et al., 2023).
GENERAL CONCLUSIONS
This focus on technology and implementation during major sporting competitions is intended to encourage further real-time monitoring innovations encompassing a wider spectrum of data. As well as helping to understand sporting performance, we highlight the potential as a preventative and recuperative telemedicine tool to inform the health of athletes during competition and potentially the wider population in the future. The lessons from monitoring of athletes can transcend sport and therefore provide a framework for remote monitoring of other populations, such as clinical patients in health-care settings or at home with chronic health issues such as diabetes, hypertension or heightened risk of falls. The use of wearable technologies transmitting numerous types of data in real time will undoubtedly become the norm at major sporting events as international sporting federations seek to make their sport more interactive and broadcast friendly. This technology has the capacity to revolutionize sport and exercise science and provides an excellent platform to understand the impact of wearable sensors on performance, wellness, health, longevity and disease.
DECLARATIONS
Acknowledgements
The following companies that have supported the development of thenecosystem for live tracking of performance and biometrics data: Human Telemtrics (London, UK), Vodafone S.A.U (Madrid, Spain), Gait Up SA (Renens, Switzerland), ARTi Analytics BV (Rotterdam, The Netherlands) and BodyCap (Caen, France). Special thanks are also extended to Mr Rafael Prada Gomez (previously Manager, Oracle Centre of Excellence at Avantic – CGI, Madrid, Spain) whose genius has been instrumental in the making and implementation of the real-time technology. The authors wish to thank the generous support and expertise provided by the following individuals throughout the course of this project; Gerasimos V Grivas, Asimina Pitsiladis, Ross Bundy, Irina Zelenkova, Kumpei Tanisawa, Hiroshi Akiyama, Fabian Schweizer, Tobias Luckfiel and Mike Miller.
FUNDING
Significant parts of the research and developments presented in this review were funded by research grants from the International Olympic Committee (Lausanne, Switzerland) and Human Telemetrics (London, UK).
CONFLICTS OF INTEREST
YP is a co-founder of Human Telemetrics (London, UK). YP is the founder of the original Sub2 marathon project now affiliated to Human Telemetrics (London, UK). The other authors have no conflicts of interest.
The views expressed are those of the authors and do not necessarily reflect the position or policy of PepsiCo, Inc.
AUTHOR AFFILIATIONS
1Department of Sport, Physical Education and Health, Hong Kong Baptist University, Hong Kong SAR
2Sports Information & External Affairs Centre, Hong Kong Sports Institute, Hong Kong
3Institute of Life and Earth Sciences, School of Energy, Geoscience, Infrastructure and Society, Heriot-Watt University, Edinburgh, UK.
4GENUD (Growth, Exercise, Nutrition and Development) Research Group, Faculty of Health and Sport Sciences, University of Zaragoza, Saragossa, Spain.
5National Observatory of Athens, Athens, Greece.
6adidas Innovation, adidas AG, Herzogenaurach, Germany.
7Environmental Stress Unit, CREPS Montpellier - Font Romeu, Montpellier, France.
8Department of Movement, Human and Health Sciences, University of Rome "Foro Italico," Rome, Italy
9European Federation of Sports Medicine Associations (EFSMA), Lausanne, Switzerland
10International Federation of Sports Medicine (FIMS), Lausanne, Switzerland
REFERENCES
Ascaso, F.J., and V. Huerva (2016). Noninvasive continuous monitoring of tear glucose using glucose-sensing contact lenses. Optom. Vis. Sci. 93:426-434.
Ash, G.I., M. Stults-Kolehmainen, M.A. Busa, A.E. Gaffey, K. Angeloudis, B. Muniz-Pardos, R. Gregory, R.A. Huggins, N.S. Redeker, S.A. Weinzimer. L.A. Grieco, K. Lyden, E. Megally, I. Vogiatzis, L. Scher, X. Zhu, J.S. Baker, C. Brandt, M.S. Businelle, L.M. Fucito, S. Griggs, R. Jarrin, B.J. Mortazavi, T. Prioleau, W. Roberts, E.K. Spanakis, L.M. Nally, A. Debruyne, N. Bachl, F. Pigozzi, F. Halabchi, D.A. Ramagole, D.C. Janse van
Rensburg, B. Wolfarth, C. Fossati, S. Rozenstoka, K. Tanisawa, M. Börjesson, J.A. Casajus, A. Gonzalez-Aguero, I. Zelenkova, J. Swart, G. Gursoy, W. Meyerson, J. Liu, D. Greenbaum, Y.P. Pitsiladis, and M.B. Gerstein (2021). Establishing a global standard for wearable devices in sport and exercise medicine: Perspectives from academic and industry stakeholders. Sports Med. 51:2237-2250.
Aughey, R.J. (2011). Applications of GPS technologies to field sports. Int. J. Sports Physiol. Perform. 6:295-310.
Baker, L.B., J. B. Model, K.A. Barnes, M.L. Anderson, S.P. Lee, K.A. Lee, S.D. Brown, A.J. Reimel, T.J. Roberts, R.P. Nuccio, J.L. Bonsignore, C.T. Ungaro, J.M. Carter, W. Li, M.S. Seib, J.T. Reeder, A.J. Aranyosi, J.A. Rogers, and R. Ghaffari (2020). Skin-interfaced microfluidic system with personalized sweating rate and sweat chloride analytics for sports science applications. Sci. Adv. 6:eabe3929.
Barnes, K.R., and A.E. Kilding (2015). Running economy: measurement, norms, and determining factors. Sport Med. Open 1:115.
Broadie, M. (2012). Assessing golfer performance on the PGA TOUR. Interfaces 42:146–165.
Buller, M., R. Fellin, M. Bursey, M. Galer, E. Atkinson, B.A. Beidleman, M.J. Marcello, K. Driver, T. Mesite, J. Seay, L. Weed, B. Telfer, C. King, R. Frazee, C. Moore, and J.R. Williamson (2022). Gait instability and estimated core temperature predict exertional heat stroke. Br. J. Sports Med. 56:446–451.
Bushnell, T., and I. Hunter (2007). Differences in technique between sprinters and distance runners at equal and maximal speeds. Sport Biomech. 6:261–268.
Clark, K.P., L.J. Ryan, and P.G. Weyand (2017). A general relationship links gait mechanics and running ground reaction forces. J. Exp. Biol. 220:247–258.
Cooper, E., A. Grundstein, A. Rosen, J. Miles, J. Ko, and P. Curry (2017). An evaluation of portable wet bulb globe temperature monitor accuracy. J. Athl. Train. 52:1161–1167.
Crang, Z.L., G. Duthie, M.H. Cole, J. Weakley, A. Hewitt, and R.D. Johnston (2021). The validity and reliability of wearable microtechnology for intermittent team sports: A systematic review. Sports Med. 51:549-565.
Dolson, C.M., E.R. Harlow, D.M. Phelan, T.J. Gabbett, B. Gaal, C. McMellen, B.J. Geletka, J.G. Calcei, J.E. Voos, D.R. Seshadri (2022). Wearable sensor technology to predict core body temperature: A systematic review. Sensors 22:7639.
Düking, P., C. Stammel, B. Sperlich, S. Sutehall, B. Muniz-Pardos, G. Lima, L. Kilduff, I. Muniz-Pardos, B., S. Sutehall, K. Angeloudis, J. Shurlock, and Y.P. Pitsiladis (2019). The use of technology to protect the health of athletes during sporting competitions in the heat. Front. Sports Act. Living 1:38.
Falbriard, M., F. Meyer, B. Mariani, G.P. Millet, and K. Aminian (2018). Accurate estimation of running temporal parameters using foot-worn inertial sensors. Front. Physiol. 9:1–10.
Falconer Hall, P., J. Blackadder-Coward, and H. Pynn (2020). Measuring wet bulb globe temperatures at point-of-exertion in worldwide UK military settings: a longitudinal observational study determining the accuracy of a portable WBGT monitor. Br. Mil. J. Mil. Health 169:161-165.
Fudge, B.W., J. Wilson, C. Easton, L. Irwin, J. Clark, O. Haddow, B. Kayser, and Y.P. Pitsiladis (2007). Estimation of oxygen uptake during fast running using accelerometry and heart rate. Med. Sci. Sports Exerc. 39:192–198.
Fuss, F.K., P. Düking, and Y. Weizman (2018). Discovery of a sweet spot on the foot with a smart wearable soccer boot sensor that maximizes the chances of scoring a curved kick in soccer. Front. Physiol. 9:63.
Fury, M.S., L.S. Oh, S.E. Linderman, J. Wright-Chisem, J.N. Fury, D.M. Scarborough, and E.M. Berkson (2021). Return to performance after ulnar collateral ligament reconstruction in major league baseball pitchers: a case-control assessment of advanced analytics, velocity, spin rates, and pitch movement. Orthop. J. Sports Med. 9:23259671211035753.
Gidley, A.D., D.E. Lankford, and J.P. Bailey (2020). The construction of common treadmills significantly affects biomechanical and metabolic variables. J. Sports Sci. 38:2236-2241.
Girard, O., F. Brocherie, J.B. Morin, and G.P. Millet. Running mechanical alterations during repeated treadmill sprints in hot versus hypoxic environments. A pilot study (2016). J. Sports Sci. 34:1190–1198.
Guppy, F., B. Muniz-Pardos, K. Angeloudis, G.V. Grivas, A. Pitsiladis, R. Bundy, I. Zelenkova, K. Tanisawa, H. Akiyama, I. Keramitsoglou, M. Miller, M. Knopp, F. Schweizer, T. Luckfiel, D. Ruiz, S. Racinais, and Y. Pitsiladis (2023). Technology innovation and guardrails in elite sport: The future is now. Sports Med. 53(Suppl 1):97–113.
Hausler, J., M. Halaki, and R. Orr (2016). Application of global positioning system and microsensor technology in competitive rugby league match-play: A systematic review and meta-analysis. Sports Med. 46:559-588.
Hill, A.V., C.N.H. Long, and H. Lupton (1924). Muscular exercise, lactic acid, and the supply and utilization of oxygen, part IV–VI. Proc. Roy. Soc. 97:84–138.
Horsley, B.J., P.J. Tofari, S.L. Halson, J.G. Kemp, J. Dickson, N. Maniar, and S.J. Cormack (2021). Does site matter? Impact of inertial measurement unit placement on the validity and reliability of stride variables during running: A systematic review and meta-analysis. Sports Med. 51:1449-1489.
Hosokawa, Y., S. Racinais, T. Akama, D. Zideman, R. Budgett, D.J. Casa, S. Bermon, A.J. Grundstein, Y.P. Pitsiladis, W. Schobersberger, and F. Yamasawa (2021). Prehospital management of exertional heat stroke at sports competitions: International Olympic Committee Adverse Weather Impact Expert Working Group for the Olympic Games Tokyo. Br. J. Sports Med. 55:1405–1410.
James, C.A., O.R. Gibson, A. Dhawan, C.M. Stewart, and A.G.B. Willmott (2021a). Volume and intensity of locomotor activity in international men's field hockey matches over a 2-year period. Front. Sports Act. Living 3:653364.
James C., A.G.B. Willmott, A. Dhawan, C. Stewart, and O.R. Gibson (2021b). Increased air temperature decreases high-speed, but not total distance, in international field hockey. Temperature 9:357-372.
Langley, J.O., and B. Langley (2023). The effect of advanced footwear technology on elite male marathon race speed. Eur. J. Appl. Physiol. (ahead of print). https://doi. org/10.1007/s00421-023-05341-x
Liu, Y., A.G. Domel, S.A. Yousefsani, J. Kondic, G. Grant, M. Zeineh, and D.B. Camarillo (2020). Validation and comparison of instrumented mouthguards for measuring head kinematics and assessing brain deformation in football impacts. Ann. Biomed. Eng. 48:2580–2598.
Longman 2016 https://www.nytimes.com/2016/05/15/sports/two-hour-marathonyannis-pitsiladis.html accessed 12th December 2023.
Lo Presti, D., C. Massaroni, P. Saccomandi, M.A. Caponero, D. Formica, and E. Schena (2017). A wearable textile for respiratory monitoring: Feasibility assessment and analysis of sensors position on system response. Annu Int Conf IEEE Eng. Med. Biol. Soc. 4423-4426.
Ma, C.Z., D.W. Wong, W.K. Lam, A.H. Wan, and W.C. Lee (2016). Balance improvement effects of biofeedback systems with state-of-the-art wearable sensors: A systematic review. Sensors 16:434.
Mariani, B., M.C. Jiménez, F.J.G. Vingerhoets, and K. Aminian (2013). On-shoe wearable sensors for gait and turning assessment of patients with Parkinson’s disease. IEEE Trans. Biomed. Eng. 60:155–58.
Moran, K., C. Richter, E. Farrell, E. Mitchell, A. Ahmadi, and N.E. O’Connor (2015). Detection of running asymmetry using a wearable sensor system. Procedia Eng. 112:180–183.
Muniz-Pardos, B., S. Sutehall, J. Gellaerts, M. Falbriard, B. Mariani, A. Bosch, M. Asrat,J. Schaible, and Y.P. Pitsiladis (2018). integration of wearable sensors into the evaluation of running economy and foot mechanics in elite runners. Curr. Sports Med. Rep. 17:480-488.
Muniz-Pardos, B., S. Sutehall, K. Angeloudis, J. Shurlock, and Y.P. Pitsiladis (2019). The use of technology to protect the health of athletes during sporting competitions in the heat. Front. Sports Act. Living 1:38.
Muniz-Pardos, B., K. Angeloudis, F.M. Guppy, K. Tanisawa, Y. Hosokawa, G. Ash, W. Schobersberger, A. Grundstein, V. Bargoria, G.O. Lwande, J.H. Ombaka, E. Ergen, F. Yamasawa, S. Racinais, D.J. Casa, and Y.P. Pitsiladis (2021a). Potential use of new cooling technologies during Tokyo 2020 Olympics and associated ethical dilemmas. Br. J. Sports Med. 55:1315-1316.
Muniz-Pardos, B., K. Angeloudis, F.M. Guppy, I. Keramitsoglou, S. Sutehall, A. Bosch, K. Tanisawa, Y. Hosokawa, G.I. Ash, W. Schobersberger, A.J. Grundstein, D.J. Casa, M.C. Morrissey, F. Yamasawa, I. Zelenkova, S. Racinais, and Y. Pitsiladis (2021b). Wearable and telemedicine innovations for Olympic events and elite sport. J. Sports Med. Phys. Fitness 61:1061–1072.
Nummela, A., T. Keränen, and L.O. Mikkelsson (2007). Factors related to top running speed and economy. Int. J. Sports Med. 28:655–661.
Nybo, L., and L. González-Alonso (2015). Critical core temperature: a hypothesis too simplistic to explain hyperthermia-induced fatigue. Scand. J. Med. Sci. Sports 25(Suppl 1):4-5.
Pigozzi, F., B. Wolfarth, A. Cintron Rodriguez, J.M. Steinacker, V. Badtieva, J.L.J. Bilzon, C. Schneider, W.O. Roberts, J. Swart, D. Constantinou, M. Dohi, T. Papadopoulou, M. Hutchinson, L. Di Luigi, M. Zahar, R. So, F.M. Guppy, J.F. Kaux, U. Madahapola, S. Rozenstoka, P. Manonelles Marqueta, J.A. Casajús, S. Racinais, K. Natsis, I. Zelenkova, B. Ulkar, E. Ozdemir, F. Arroyo, A. Pedrinelli, M. Miller, N. Bachl, M. Geistlinger, and Y.P. Pitsiladis (2021). Protecting Olympic participants from COVID-19: The trialled and tested process. Br. J. Sports Med. 55:1322-1323.
Pino-Ortega, J., A. Bastida-Castillo, C. Gómez-Carmona, and M. Rico-González (2020). Validity and reliability of an eight antennae ultra-wideband local positioning system to measure performance in an indoor environment. Sports Biomech. DOI: 10.1080/14763141.2020.1830162.
Polar (2023). https://www.polar.com/blog/40-years-of-incredible-firsts-polar-history/Acessed 12th December 2023.
Ponce-Bordón, J.C., D. Lobo-Triviño, A. Rubio-Morales, R. López Del Campo, R. Resta, and M.A. López-Gajardo (2022). The effect of the video assistant referee system implementation on match physical demands in the Spanish LaLiga. Int. J. Environ. Res. Public Health 19:5125.
Pugh, L.G. (1958). Muscular exercise on Mount Everest. J. Physiol. 141:233–261. Racinais, S., S. Moussay, D. Nichols, G. Travers, T. Belfekih, Y.O. Schumacher, and J.D. Periard (2019). Core temperature up to 41.5ºC during the UCI Road Cycling World Championships in the heat. Br. J. Sports Med. 53:426-429.
Racinais, S., G. Havenith, P. Aylwin, M. Ihsan, L. Taylor, P.E. Adami, M.C. Adamuz, M. Alhammoud. J.M. Alonso,, N. Bouscaren, S. Buitrago, M. Cardinale, N. van Dyk, C.J. Esh, J. Gomez-Ezeiza, F. Garrandes, L. Holtzhausen, M. Labidi, G. Lange, A. Lloyd, S. Moussay, K. Mtibaa, N. Townsend, M.G. Wilson, and S. Bermon (2022).
Association between thermal responses, medical events, performance, heat acclimation and health status in male and female elite athletes during the 2019 Doha World Athletics Championships. Br. J. Sports Med. 56:439–445.
Racinais, S., Y. Hosokawa, T. Akama, S. Bermon, X. Bigard, D.J. Casa, A. Grundstein, O. Jay, A. Massey, S. Migliorini, M. Mountjoy, N. Nikolic, Y.P. Pitsiladis, W. Schobersberger, J.M. Steinacker, F. Yamasawa, D.A. Zideman, L. Engebretsen, and R. Budgett (2023). IOC consensus statement on recommendations and regulations for sport events in the heat. Br. J. Sports Med. 57:8-25.
Robertson, J., K. Zendler, J. De Mey, G.I. Haycraft, C. Ash, D. Brockett, C. Seshadri, L. Woods, R. Kober, J. Aughey and J. Rogowski (2024). Development of a sports technology quality framework, J. Sports Sci. (ahead of print), DOI: 10.1080/02640414.2024.2308435.
Santos-Concejero, J., N. Tam, C. Granados, J. Irazusta, I. Bidaurrazaga-Letona, J. Zabala-Shei, R.J., I.G. Holder, A.S. Oumsang, B.A. Paris, and H.L. Paris (2022). Wearable activity trackers-advanced technology or advanced marketing? Eur. J. Appl. Physiol. 122:1975- 1990.
Seçkin, A.Ç., S.B. Ate, and M. Seçkin (2023). Review on wearable technology in sports: Concepts, challenges and opportunities. Appl. Sci. 13:10399. Tartaruga, M.P., J. Brisswalter, L.A. Peyré-Tartaruga, A.O. Avila, C.L. Alberton, M. Coertjens, E.L. Cadore, C.L. Tiggemann, E.M. Silva, and L.F. Kruel (2012). The relationship between running economy and biomechanical variables in distance runners. Res. Q. Exerc. Sport. 83:367– 375.
Tonekaboni, N.H., A. Grundstein, L. Ramaswamy, S. Kulkarni, D. Mishra, and Y. Yin (2018). Scouts: A smart community centric urban heat monitoring framework. In: Proceedings of the 1st ACM SIGSPATIAL international workshop on advances in resilient and intelligent cities, ARIC 2018, pp 27–30. Tour de France 2022 – The technology behind the world’s largest connected stadium. https:// services.global.ntt/en-au/tourdefrance. Accessed: June 23rd 2023.
Wickham, H., M. Averick, J. Bryan, W. Chang, L.D. McGowan, R. François, G. Grolemund, A. Hayes, L. Henry, J. Hester, M. Kuhn, T.L. Pedersen, E. Miller, S.M. Bache, K. Müller, J. Ooms, D. Robinson. D.P. Seidel, V. Spinu, K. Takahashi, D. Vaughan, C. Wilke, K. Woo, and H. Yutani (2019). Welcome to the tidyverse. J. Open Source Software 4:1686.
Willmott A., C.A. James, A. Bliss, R.A. Leftwich, and N.S. Maxwell (2019). A comparison of two global positioning system devices for team-sport running protocols. J. Biomech. 83:324-328.