KEY POINTS
- Fatigue during ~1-10 min of high-intensity sports/events is multifactorial, but there are strong mechanistic underpinnings demonstrating skeletal muscle acidosis, via accumulating hydrogen ion (H+), to be a key performance limiter. Accordingly, skeletal muscle has various innate intra- and extra-cellular buffering mechanisms to address exercise-induced acidosis.
- Carnosine is a key intra-cellular buffer due to its nitrogen containing imidazole side ring, which can accept (buffer) H+ and slow the decline in muscle pH during intense exercise and contribute as much as ~15% to total buffering capacity.
- Beyond its role in buffering, carnosine has also been shown to be a diffusible calcium (Ca2+)/H+ exchanger, delivering Ca2+ back to the sarcoplasmic reticulum and H+ away to the cell membrane, suggesting that it might also enhance muscle Ca2+ sensitivity and contraction efficiency.
- Carnosine in muscle is synthesized by carnosine synthase, in which the plasma beta-alanine concentration is the rate limiting substrate; consistent data has demonstrated that ~3-6 g beta-alanine daily over at least 4 weeks of supplementation can augment muscle carnosine stores by 30-60%.
- Several meta-analyses have shown moderate effect sizes for exercise capacity, and smaller effect sizes for exercise performance over ~1-10 min duration. This translates into ~2-3 % performance benefits in non-elite subjects, but ~0.5-1% increases in elite subjects. However, more data is required in elite athlete cohorts.
- Despite significant increases in scientific knowledge regarding beta-alanine supplementation protocols and performance efficacy since 2006, there are many prevailing questions and future applied research directions that remain.
INTRODUCTION
Athletes participating in high-intensity sports/events (~1-10 min of all-out effort), or in sports where athletes are required to make repeated high-intensity efforts, have unique performance determinants. All of these sports use large amounts of anaerobically derived adenosine triphosphate (ATP) energy production from phosphocreatine and anaerobic glycolysis, the latter resulting in large amounts of lactate accumulation (>10 mmol/L). The diversity of these unique high-intensity performance determinates is especially prevalent in the middle-distance/high-intensity based events, which feature a blend of aerobic, anaerobic and neuromuscular/mechanical characteristics (Sandford & Stellingwerff, 2019) and many of these determinates are linked to nutritional interventions (Stellingwerff, Bovim, & Whitfield, 2019). Indeed, the limits to high-intensity performance are multifactorial, but a major limiting factor is the ability to tolerate ever increasing levels of muscle acidosis; both intra- and extra-cellular. To enhance extra-cellular buffering, sodium-bicarbonate loading has been researched and utilized by athletes for many decades.
However, it was only in the mid-2000’s that pioneering work by Prof. Roger Harris and colleagues (2006) demonstrated that augmenting intra-cellular (within muscle) buffering was also possible via chronic (several weeks) beta-alanine supplementation, which significantly increased muscle carnosine (b-alanyl-L-histidine) and high-intensity performance (Hill et al., 2007) (Figure 1). Since this time, there has been an explosion of research examining the efficacy of beta-alanine supplementation to optimally augment the muscle carnosine content and enhance subsequent performance. Therefore, this Sports Science Exchange (SSE) article will first examine the limitations to high-intensity performance, with emphasis on anaerobic metabolism and associated buffering mechanisms, and then examine the science of beta-alanine supplementation protocols and various performance outcomes.
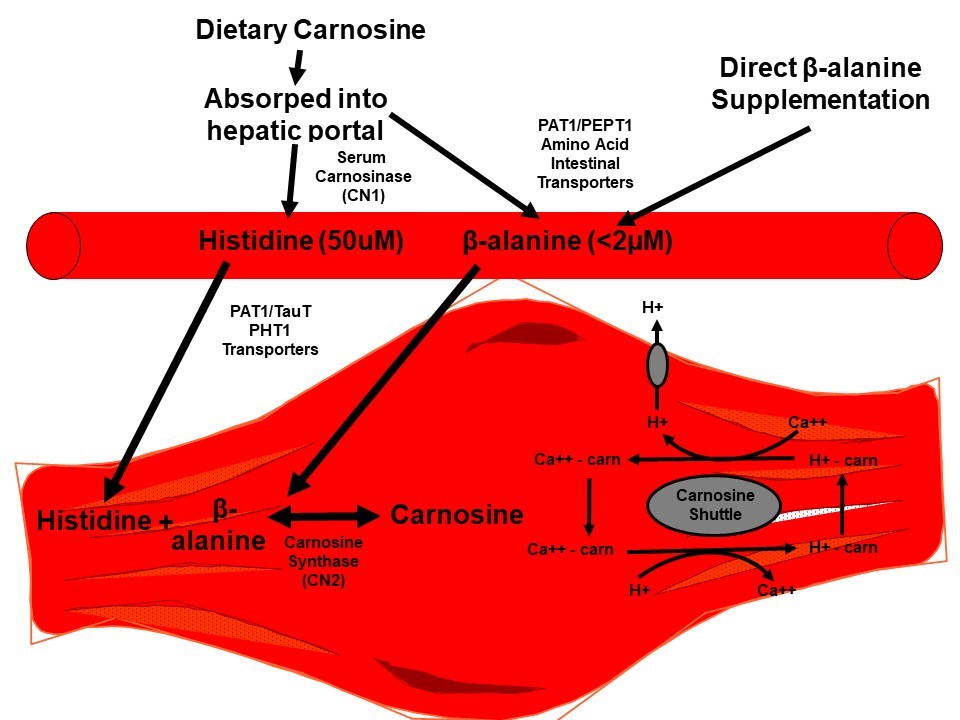
LIMITATIONS TO HIGH-INTENSITY EXERCISE PERFORMANCE AND MUSCLE CARNOSINE MECHANISMS OF ACTION
High-intensity sports/events (~1-10 min of all-out effort) are unique since they are at the crossroads of metabolism and receive large contributions of energy from aerobic and anaerobic metabolism (Figure 2). There are three primary energy systems that simultaneously (although at differing rates) provide ATP to power high-intensity exercise, namely the anaerobic systems (also named substrate level phosphorylation): 1) phosphocreatine (PCr) degradation and 2) “anaerobic” glycolysis, and 3) aerobic metabolism (also named oxidative phosphorylation). During the onset of exercise and during exercise situations with increasing intensity, ATP production from aerobic metabolism cannot match the rate of ATP utilization (100% of high-intensity events), and the shortfall in energy supply is made up by anaerobic metabolism. This energy is provided by the PCr and anaerobic glycolysis systems. The latter has a larger capacity than PCr degradation and provides ATP primarily via the breakdown of glycogen in the glycolytic pathway, resulting in excessive pyruvate production. During extreme intensity exercise the high rate of pyruvate production exceeds the rate at which it can be aerobically oxidized by pyruvate dehydrogenase in the mitochondria via the tricarboxylic acid cycle and electron transport chain (Spriet, Howlett, & Heigenhauser, 2000), leading to the extreme levels of lactate production associated with high-intensity sports (competition and training).
Accordingly, high-intensity efforts lasting ~4 min are conducted at ~20-times resting VO2 values with resultant blood lactate as high as ~25 mmol/L, and the production of hydrogen ions (H+) causing decreases in human skeletal muscle pH from ~7.2 at rest to 6.6 at exhaustion (Hermansen & Osnes, 1972). Although fatigue is multifactorial, there are certainly strong mechanistic underpinnings demonstrating muscle acidosis, via accumulating H+, to be a key limiter to sustained high-intensity exercise over ~1-10 min (Allen, Lamb, & Westerblad, 2008). It has also been known for 80+ years that carnosine is a key intra-cellular buffer due to its nitrogen containing imidazole side ring, which can accept (buffer) H+ (Bate-Smith, 1938), and slow the decline in muscle pH during intense exercise (Baguet, Koppo, Pottier, & Derave, 2010). The contribution of normal muscle carnosine levels to total intracellular muscle buffering capacity has been suggested to be ~6-7%, but can reach ~15% of total when augmented via beta-alanine supplementation (Harris & Stellingwerff, 2013). Interestingly, it has also been known for 35 years that sprinters and rowers have nearly double the amount of muscle carnosine than marathon runners, and carnosine strongly correlates with type II muscle fiber content (Parkhouse, McKenzie, Hochachka, & Ovalle, 1985). Taken together, severe metabolic acidosis is a limiter to high-intensity performance of which muscle carnosine can act as one of many intracellular buffers.
Beyond metabolic acidosis limiting ATP production, there are also biomechanical and structural constraints to high-intensity performance that are often not considered when elucidating the limits to high-intensity performance. For example, many middle-distance/power based sports require extreme morphological adaptations (e.g., exceptional quad girths in sprint cycling and rowing) that result in extraordinary speed and explosive strength and/or power. Obviously performance determinants of these sports also need to be considered from a structural and biomechanical origin (e.g., absolute peak force, rate of force development, body mass, etc. (Weyand, Sandell, Prime, & Bundle, 2010)). Near maximal sustained high-intensity running speed appears to be limited by the minimum time needed (ground contact time or time under tension) to apply large forces, which are again related to fast-twitch type II muscle fiber content (Weyand et al., 2010) and/or the ability of the muscle to repeatedly generate maximal force. Underpinning these various structural/mechanical determinants of performance are fast-twitch Type II muscle fibers (Type IIa and IIx) (Costill et al., 1976)), which have nearly double the muscle carnosine content compared to slow-twitch Type I muscle fibers (Baguet et al., 2011; Parkhouse et al., 1985; Stellingwerff, Anwander, et al., 2012).
Carnosine also has numerous proposed physiological roles, although most of the mechanistic support has been generated via cell-culture and rodent research. Nevertheless, many of these in vitro roles may be at play during rest and exercise in humans as well. All of these potential physiological roles have recently been eloquently reviewed by Matthews et al. (2019) with the strongest prevailing ergogenic mechanism for augmented carnosine in athletes being enhanced intra-cellular pH buffering. Secondary mechanisms also include: (1) calcium (Ca2+) handling, including release, reuptake and Ca2+ sensitivity; (2) cytoplasmic Ca2+ - H+ exchanger described as the “carnosine shuttle” (Figure 1); (3) potential to regulate bioenergetics leading to an increase in glycolytic flux; (4) scavenger of reactive oxygen species altering oxidative stress; and (5) formation of stable conjugates to prevent reactive aldehydes and lipid peroxidation (non-enzymatic detoxification of reactive aldehydes) from occurring.
An interesting emerging mechanism is the carnosine shuttle hypothesis (Figure 1). In support of the carnosine shuttle hypothesis, recent data in cardiac myocytes has demonstrated that carnosine is not just a buffer, but is also involved in Ca2+ and H+ handling from the sarcoplasmic reticulum as a type of “carnosine shuttle” (Figure 1; as reviewed by (Blancquaert, Everaert, & Derave, 2015; Matthews, Artioli, Turner, & Sale, 2019), but requires confirmation in human skeletal muscle.
BETA-ALANINE SUPPLEMENTATION AND CARNOSINE SYNTHESIS
Carnosine in muscle is synthesized by carnosine synthase, in which the plasma beta-alanine concentration is the rate limiting substrate. The plasma concentration is <2 μM and the Km (concentration needed for a reaction rate that is 50% of maximum) for its uptake into muscle is ~1.0–2.3 mM). In contrast, L-histidine is present in much higher concentrations in plasma (50 μM) and muscle and has a much lower Km (16.8 μM, Figure 1). In 2006, Harris and colleagues (2006) were the first to demonstrate that oral ingestion of 3.2 or 6.4 g/day of beta-alanine (or an isomolar amount of supplemented carnosine (13.0 g/day)) resulted in a 40-60% increase in muscle carnosine in humans.
Since this landmark study, every study that has measured muscle carnosine (either via muscle biopsy or magnetic resonance spectroscopy), where a significant amount of beta-alanine was supplemented (e.g. ~3-6 g beta-alanine/day over at least 4 weeks), has shown a significant increase in muscle carnosine content (for reviews see,(Perim et al., 2019; Stellingwerff, Decombaz, Harris, & Boesch, 2012). On average, this has led to a significant ~30-50% increase in muscle carnosine content with ~3-6 g beta-alanine supplemented/day over 4-6 weeks. The washout of augmented skeletal muscle carnosine after the termination of beta-alanine supplementation is very slow, with an estimated washout time of ~14 to 15 weeks after a ~50% increase in muscle carnosine (Baguet et al., 2009; Stellingwerff, Anwander, et al., 2012). By far the biggest contributing factor to carnosine synthesis is the dose and duration of beta-alanine supplementation, as studies have shown that doubling the daily dose of beta-alanine results in about double the amount of carnosine synthesized (and halves the time to get to the same increase) (Church et al., 2017; Stellingwerff, Anwander, et al., 2012).
Contemporary data has also shown several potential ways to increase the efficiency of carnosine synthesis with beta-alanine supplementation, as only ~5% of ingested beta-alanine can be accounted for in muscle as carnosine, with the rest having an unknown metabolic fate. These include: (1) taking beta-alanine with a meal (providing an insulin effect), as this resulted in greater carnosine synthesis in the soleus, but not gastrocnemius muscle (Stegen et al., 2013); (2) using slow-release beta-alanine as, compared to the pure form, results in greater carnosine synthesis (Varanoske et al., 2019); and (3) undertaking training/exercise while supplementing (Bex, Chung, Baguet, Achten, & Derave, 2015). However, beyond dose and duration of beta-alanine supplementation to impact on carnosine synthesis, these other influencing factors require more scientific validation. Furthermore, a recent seminal study by Saunders et al, (2017a) which implemented 24 weeks of beta-alanine supplementation at 6.4 g/day with 25 subjects, reported a huge range of individual variability for carnosine synthesis. Five subjects had maximal muscle carnosine contents at week 24, but another 5 subjects already had their peak muscle carnosine content within the first 12 weeks (Saunders, Painelli, et al., 2017). Finally, it does appear that once muscle carnosine is elevated with a loading phase of beta-alanine, continued supplementation with as little as 1.2 g/day of beta-alanine will maintain the elevated carnosine stores (Stegen et al., 2014). Obviously, our understanding of the determinants of the individual response to beta-alanine supplementation are far from being completely understood (Table 1).
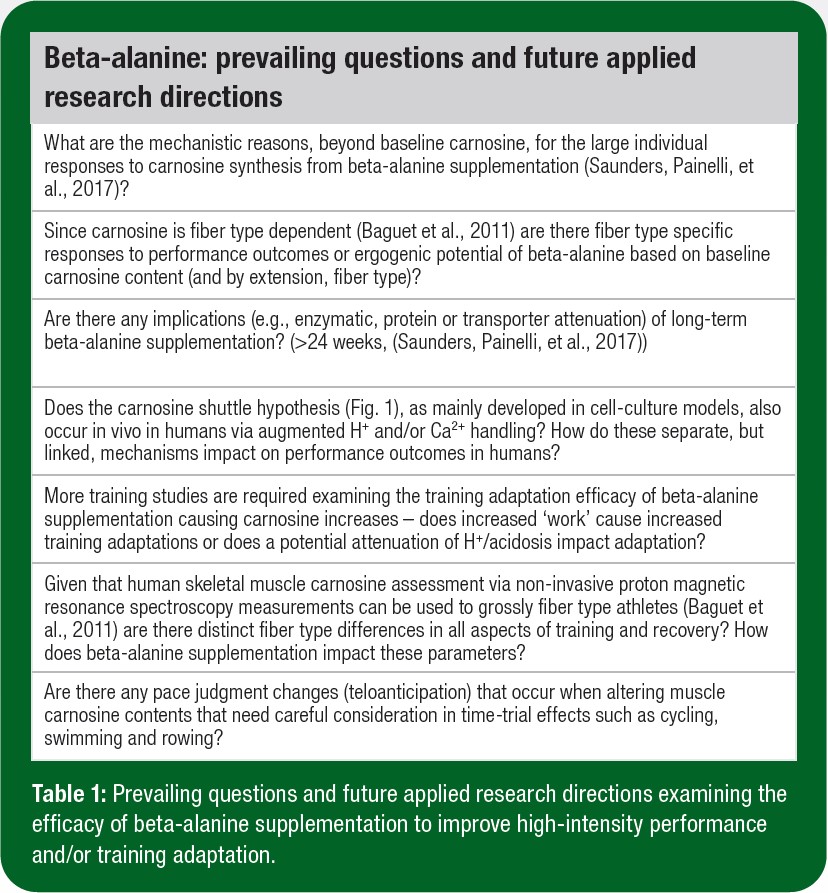
When beta-alanine is supplemented at levels greater than 800 mg/dose it results in mild paresthesia in most subjects (minor “pins and needles,” skin vasodilation and flushing over ~60-120 min). However, a commercialized slow release beta-alanine tablet has been produced and demonstrated less urinary spillover and a blunting of the peak plasma curve (same area under the curve), with none of the subjects reported any paresthesia symptoms as compared to control (Decombaz, Beaumont, Vuichoud, Bouisset, & Stellingwerff, 2012). Other than a downregulation of the beta-alanine muscle transporter gene and its subsequent protein TauT (Saunders, Painelli, et al., 2017), no other side effects or changes to any blood measurements or acute body weight changes have been demonstrated with beta-alanine supplementation (Harris et al., 2006; Stellingwerff, Anwander, et al., 2012).
PERFORMANCE OUTCOMES OF AUGMENTED CARNOSINE VIA BETA-ALANINE SUPPLEMENTATION
The first beta-alanine human performance study was published in 2007 and demonstrated that an increase in muscle carnosine (via beta-alanine supplementation) resulted in dose-response increases in total work done time to exhaustion (TTE) at 110% of wattage maximum (Wmax) at week 4 (+12% TTE) and week 10 (+16% TTE) compared to baseline (Hill et al., 2007). In the following years, a plethora of performance-based studies have demonstrated the efficacy of beta-alanine supplementation to enhance high-intensity outcomes, including: (1) pre-supplementation baseline muscle carnosine being correlated to ~16 s, ~1.5 min, and ~6.5 min rowing performance (Baguet, Bourgois, Vanhee, Achten, & Derave, 2010), showing the importance of muscle carnosine to high-intensity performance; (2) significantly improved 30 s sprint performance (+5% mean power) after prolonged 110 min of cycling (Van Thienen et al., 2009); and (3) enhanced repeated Wingate outcomes (total work done over 4 Wingate tests) in both trained and untrained subjects (de Salles Painelli et al., 2014). However, some studies have demonstrated no performance benefits after beta-alanine supplementation as compared to control, but generally with either supplementation featuring less than ~150 g of total supplemented beta-alanine (Derave et al., 2007; Smith et al., 2009) or with very short, explosive performance protocols under ~30 sec of duration (e.g., 1 repetition maximum, or sprinting), where acidosis will not be limiting (Kendrick et al., 2008; Sweeney, Wright, Glenn Brice, & Doberstein, 2010).
There have been two definitive meta-analyses examining the performance effects of chronic beta-alanine supplementation. The first meta-analysis was published in 2012 and featured 15 studies (n=360 subjects) with an overall outcome of beta-alanine improving the exercise outcome measures compared to control (p=0.002 with an overall effect size (ES) of 0.374) and the majority of the performance effects being demonstrated over 1 to 4 min of high-intensity exercise (Hobson, Saunders, Ball, Harris, & Sale, 2012). However, this meta-analysis was dominated by exercise capacity/TTE protocols (n=13 studies; p=0.013, ~2-3% improvement) compared to exercise performance/time-trial (TT) protocols (n=2 studies; p=0.204). It is important to note that effect sizes tend to be greater in duration based TTE protocols, as compared to TTs where intrinsic pacing can also play a role in performance outcomes. A follow-up meta-analysis was completed in 2017 featuring 40 studies (n=1461 subjects), where the overall effect size was 2.1-times smaller (ES=0.18) compared to the 2012 meta-analysis (Saunders, Elliott-Sale, et al., 2017). Interestingly, the ES for exercise capacity/TTE was 0.4998, which was similar to the 2012 meta-analysis, but the ES for performance/TT outcomes was only 0.1078. Again, meta-regression analysis showed that exercise duration of ~1-10 min demonstrated the greatest performance benefits with beta-alanine supplementation (p=0.004; Figure 2).
Finally, there has been some emerging data suggesting smaller overall performance effects (~0.5-1% improvement in performance) of beta-alanine supplementation in well-trained subjects compared to untrained subjects (Bellinger, Howe, Shing, & Fell, 2012; Saunders, Elliott-Sale, et al., 2017). This has been demonstrated in other meta-analyses in the past and probably represents a biological performance ceiling effect found with elite athletes approaching a genetic zenith. Figure 2 highlights the various energy systems, and the performance “sweet spot” of ~1-10 min of duration and the various Olympic sports that, accordingly, might benefit from beta-alanine supplementation. Beta-alanine is one of a few ergogenic aids to be listed as potentially ergogenic (when used with the correct protocol in ideal events/sports) as part of the recent International Olympic Committee supplement consensus (Peeling, Binnie, Goods, Sim, & Burke, 2018).
PRACTICAL APPLICATIONS & CONCLUSIONS
Since Harris and colleagues first showed in 2006 that chronic beta-alanine supplementation significantly increased muscle carnosine contents in humans there have been large advances in our knowledge regarding supplementation protocols and subsequent human performance outcomes. Nevertheless, Table 1 highlights some of our prevailing questions and future applied research directions.
Beyond these outstanding limitations in understanding, the current contemporary practical applications and recommendations for the supplementation of beta-alanine include:
- Data supports taking ~3-6 g beta-alanine/day (usually take 800 mg to 1.6 g doses every 4 hours with a meal) over at least 4 weeks of supplementation to augment carnosine stores (30-60%) and demonstrate performance benefits.
- There is some evidence that this dosing protocol can be optimized to body weight (~0.05-0.06 g/kg body mass/day) and that slow-release beta-alanine, taken with food and exercise will further enhance carnosine synthesis (Stegen et al., 2014). However, at 24 weeks of supplementation, some athletes may still be increasing their muscle carnosine content.
- Two meta-analyses have supported that high-intensity sports/events (~1-10 min in duration, with significantly high blood lactate levels (>10 mmol/L, Figure 2)) appear to have the most ergogenic potential from the supplementation of beta-alanine.
- Small to moderate performance effects have been reported with beta-alanine in high-intensity sustained events, with 2-3% increases in performance in recreational athletes and ~0.5-1.0% in elite athletes.
This SSE provides a contemporary update on the applied science regarding beta-alanine supplementation and its impact on muscle carnosine content and exercise performance outcomes. Although many questions remain (Table 1), the explosion of knowledge in the last 15 years has made beta-alanine one of a handful of supplements with ergogenic potential when utilized with an optimal dosing protocol in sustained high-intensity events.
The views expressed are those of the authors and do not necessarily reflect the position or policy of PepsiCo, Inc.
REFERENCES
Allen, D. G., Lamb, G. D., & Westerblad, H. (2008). Skeletal muscle fatigue: cellular mechanisms. Physiological reviews, 88(1), 287-332. doi:10.1152/physrev.00015.2007
Baguet, A., Bourgois, J., Vanhee, L., Achten, E., & Derave, W. (2010). Important role of muscle carnosine in rowing performance. Journal of Applied Physiology, 109(4), 1096-1101. doi:10.1152/japplphysiol.00141.2010
Baguet, A., Everaert, I., Hespel, P., Petrovic, M., Achten, E., & Derave, W. (2011). A new method for non-invasive estimation of human muscle fiber type composition. PLoS ONE, 6(7), e21956. doi:10.1371/journal.pone.0021956
Baguet, A., Koppo, K., Pottier, A., & Derave, W. (2010). Beta-alanine supplementation reduces acidosis but not oxygen uptake response during high-intensity cycling exercise. European Journal of Applied Physiology, 108(3), 495-503. doi:10.1007/s00421-009-1225-0
Baguet, A., Reyngoudt, H., Pottier, A., Everaert, I., Callens, S., Achten, E., & Derave, W. (2009). Carnosine loading and washout in human skeletal muscles. Journal of Applied Physiology, 106(3), 837-842. doi:10.1152/japplphysiol.91357.2008
Bate-Smith, E. C. (1938). The buffering of muscle in rigor: protein, phosphate and carnosine. Journal of Physiology, 92(3), 336-343.
Bellinger, P. M., Howe, S. T., Shing, C. M., & Fell, J. W. (2012). Effect of combined beta-alanine and sodium bicarbonate supplementation on cycling performance. Medicine and science in sports and exercise, 44(8), 1545-1551. doi:10.1249/MSS.0b013e31824cc08d
Bex, T., Chung, W., Baguet, A., Achten, E., & Derave, W. (2015). Exercise training and beta-alanine-induced muscle carnosine loading. Frontiers in Nutrition, 2, 13. doi:10.3389/fnut.2015.00013
Blancquaert, L., Everaert, I., & Derave, W. (2015). Beta-alanine supplementation, muscle carnosine and exercise performance. Current opinion in clinical nutrition and metabolic care, 18(1), 63-70. doi:10.1097/MCO.0000000000000127
Church, D. D., Hoffman, J. R., Varanoske, A. N., Wang, R., Baker, K. M., La Monica, M. B., . . . Stout, J. R. (2017). Comparison of two beta-alanine dosing protocols on muscle carnosine elevations. Journal of the American College of Nutrition, 36(8), 608-616. doi:10.1080/07315724.2017.1335250
Costill, D. L., Daniels, J., Evans, W., Fink, W., Krahenbuhl, G., & Saltin, B. (1976). Skeletal muscle enzymes and fiber composition in male and female track athletes. Journal of Applied Physiology, 40(2), 149-154. doi:10.1152/jappl.1976.40.2.149
de Salles Painelli, V., Saunders, B., Sale, C., Harris, R. C., Solis, M. Y., Roschel, H., . . . Lancha, A. H., Jr. (2014). Influence of training status on high-intensity intermittent performance in response to beta-alanine supplementation. Amino Acids, 46(5), 1207-1215. doi:10.1007/s00726-014-1678-2
Decombaz, J., Beaumont, M., Vuichoud, J., Bouisset, F., & Stellingwerff, T. (2012). Effect of slow-release beta-alanine tablets on absorption kinetics and paresthesia. Amino Acids, 43(1), 67-76. doi:10.1007/s00726-011-1169-7
Derave, W., Ozdemir, M. S., Harris, R. C., Pottier, A., Reyngoudt, H., Koppo, K., . . . Achten, E. (2007). beta-Alanine supplementation augments muscle carnosine content and attenuates fatigue during repeated isokinetic contraction bouts in trained sprinters. Journal of Applied Physiology, 103(5), 1736-1743. doi:10.1152/japplphysiol.00397.2007
Harris, R. C., & Stellingwerff, T. (2013). Effect of beta-alanine supplementation on high-intensity exercise performance. Nestle Nutrition Institute Workshop Series, 76, 61-71. doi:10.1159/000350258
Harris, R. C., Tallon, M. J., Dunnett, M., Boobis, L., Coakley, J., Kim, H. J., . . . Wise, J. A. (2006). The absorption of orally supplied beta-alanine and its effect on muscle carnosine synthesis in human vastus lateralis. Amino Acids, 30(3), 279-289.
Hermansen, L., & Osnes, J. B. (1972). Blood and muscle pH after maximal exercise in man. Journal of Applied Physiology, 32(3), 304-308. doi:10.1152/jappl.1972.32.3.304
Hill, C. A., Harris, R. C., Kim, H. J., Harris, B. D., Sale, C., Boobis, L. H., . . . Wise, J. A. (2007). Influence of beta-alanine supplementation on skeletal muscle carnosine concentrations and high intensity cycling capacity. Amino Acids, 32(2), 225-233. doi:10.1007/s00726-006-0364-4
Hobson, R. M., Saunders, B., Ball, G., Harris, R. C., & Sale, C. (2012). Effects of beta-alanine supplementation on exercise performance: a meta-analysis. Amino Acids, 43(1), 25-37. doi:10.1007/s00726-011-1200-z
Kendrick, I. P., Harris, R. C., Kim, H. J., Kim, C. K., Dang, V. H., Lam, T. Q., . . . Wise, J. A. (2008). The effects of 10 weeks of resistance training combined with beta-alanine supplementation on whole body strength, force production, muscular endurance and body composition. Amino Acids, 34(4), 547-554. doi:10.1007/s00726-007-0008-3
Matthews, J. J., Artioli, G. G., Turner, M. D., & Sale, C. (2019). The physiological roles of carnosine and beta-alanine in exercising human skeletal muscle. Medicine and science in sports and exercise, 51(10), 2098-2108. doi:10.1249/MSS.0000000000002033
Parkhouse, W. S., McKenzie, D. C., Hochachka, P. W., & Ovalle, W. K. (1985). Buffering capacity of deproteinized human vastus lateralis muscle. Journal of Applied Physiology, 58(1), 14-17. doi:10.1152/jappl.1985.58.1.14
Peeling, P., Binnie, M. J., Goods, P. S. R., Sim, M., & Burke, L. M. (2018). Evidence-based supplements for the enhancement of athletic performance. International Journal of Sport Nutrition and Exercise Metabolism, 28(2), 178-187. doi:10.1123/ijsnem.2017-0343
Perim, P., Marticorena, F. M., Ribeiro, F., Barreto, G., Gobbi, N., Kerksick, C., . . . Saunders, B. (2019). Can the skeletal muscle carnosine response to beta-alanine supplementation be optimized? Frontiers in Nutrition, 6, 135. doi:10.3389/fnut.2019.00135
Sandford, G. N., & Stellingwerff, T. (2019). ‘Question your categories’: the misunderstood complexity of middle-distance running profiles with implications for research methods and application. Front. Sports Act. Living. doi:https://doi.org/10.3389/fspor.2019.00028
Saunders, B., Elliott-Sale, K., Artioli, G. G., Swinton, P. A., Dolan, E., Roschel, H., . . . Gualano, B. (2017). beta-alanine supplementation to improve exercise capacity and performance: a systematic review and meta-analysis. British Journal of Sports Medicine, 51(8), 658-669. doi:10.1136/bjsports-2016-096396
Saunders, B., Painelli, V. D. S., Oliveira, L. F., Silva, V. D. E., Silva, R. P., Riani, L., . . . Gualano, B. (2017). Twenty-four Weeks of beta-Alanine Supplementation on Carnosine Content, Related Genes, and Exercise. Medicine and science in sports and exercise, 49(5), 896-906. doi:10.1249/MSS.0000000000001173
Smith, A. E., Moon, J. R., Kendall, K. L., Graef, J. L., Lockwood, C. M., Walter, A. A., . . . Stout, J. R. (2009). The effects of beta-alanine supplementation and high-intensity interval training on neuromuscular fatigue and muscle function. European Journal of Applied Physiology, 105(3), 357-363. doi:10.1007/s00421-008-0911-7
Spriet, L. L., Howlett, R. A., & Heigenhauser, G. J. (2000). An enzymatic approach to lactate production in human skeletal muscle during exercise. Medicine and science in sports and exercise, 32(4), 756-763. doi:10.1097/00005768-200004000-00007
Stegen, S., Bex, T., Vervaet, C., Vanhee, L., Achten, E., & Derave, W. (2014). beta-Alanine dose for maintaining moderately elevated muscle carnosine levels. Medicine and science in sports and exercise, 46(7), 1426-1432. doi:10.1249/MSS.0000000000000248
Stegen, S., Blancquaert, L., Everaert, I., Bex, T., Taes, Y., Calders, P., . . . Derave, W. (2013). Meal and beta-alanine coingestion enhances muscle carnosine loading. Medicine and science in sports and exercise, 45(8), 1478-1485. doi:10.1249/MSS.0b013e31828ab073
Stellingwerff, T., Anwander, H., Egger, A., Buehler, T., Kreis, R., Decombaz, J., & Boesch, C. (2012). Effect of two beta-alanine dosing protocols on muscle carnosine synthesis and washout. Amino Acids, 42(6), 2461-2472. doi:10.1007/s00726-011-1054-4
Stellingwerff, T., Bovim, I. M., & Whitfield, J. (2019). Contemporary nutrition interventions to optimize performance in middle-distance runners. International journal of sport nutrition and exercise metabolism, 29(2), 106-116. doi:10.1123/ijsnem.2018-0241
Stellingwerff, T., Decombaz, J., Harris, R. C., & Boesch, C. (2012). Optimizing human in vivo dosing and delivery of beta-alanine supplements for muscle carnosine synthesis. Amino Acids, 43(1), 57-65. doi:10.1007/s00726-012-1245-7
Sweeney, K. M., Wright, G. A., Glenn Brice, A., & Doberstein, S. T. (2010). The effect of beta-alanine supplementation on power performance during repeated sprint activity. Journal of strength and conditioning research / National Strength & Conditioning Association, 24(1), 79-87. doi:10.1519/JSC.0b013e3181c63bd5
Van Thienen, R., Van Proeyen, K., Vanden Eynde, B., Puype, J., Lefere, T., & Hespel, P. (2009). Beta-alanine improves sprint performance in endurance cycling. Medicine and science in sports and exercise, 41(4), 898-903. doi:10.1249/MSS.0b013e31818db708
Varanoske, A. N., Hoffman, J. R., Church, D. D., Coker, N. A., Baker, K. M., Dodd, S. J., . . . Stout, J. R. (2019). Comparison of sustained-release and rapid-release beta-alanine formulations on changes in skeletal muscle carnosine and histidine content and isometric performance following a muscle-damaging protocol. Amino Acids, 51(1), 49-60. doi:10.1007/s00726-018-2609-4
Weyand, P. G., Sandell, R. F., Prime, D. N., & Bundle, M. W. (2010). The biological limits to running speed are imposed from the ground up. Journal of Applied Physiology (1985), 108(4), 950-961. doi:10.1152/japplphysiol.00947.2009