KEY POINTS
- Athletes need to pay attention to the three “R’s” after exercise – rehydrate, replenish (muscle glycogen), and repair (damaged proteins). Effective adaptation to training will occur only if all three R’s are attended to.
- Protein ingestion and resistance exercise both stimulate the process of new muscle protein synthesis (MPS) and are synergistic when protein consumption follows exercise. In healthy persons, changes in MPS are much greater in their influence over net muscle gain than changes in muscle protein breakdown (MPB).
- Summation of positive periods of muscle protein balance (i.e., when MPS exceeds MPB) leads to muscle hypertrophy. Consumption of protein in close temporal proximity, with evidence currently favoring consumption of protein after exercise, leads to greater rates of MPS and greater hypertrophy.
- The exact period of time after exercise during which it would be beneficial to consume protein is not known; however, the anabolic effect of exercise is long-lasting, but likely diminishes with increasing time post-exercise.
- For the maintenance and building of muscle mass a higher overall daily protein intake (greater than the RDA, which currently stands at 0.8 g protein/kg body mass/d) in the range of 1.4-1.6 g/kg/d should be consumed.
- Athletes should consume protein as part of each meal to promote optimal protein synthesis following each meal (0.25-0.30 g protein/kg body mass/meal). Additionally, high-quality proteins, such as milk-based proteins like casein and whey, can spare lean mass losses and can promote gains in lean mass during periods of intense training or caloric restriction.
- Rapidly digested proteins that contain high proportions of essential amino acids (EAA), of which the key amino acid appears to be leucine, are more effective in stimulating MPS than other proteins.
- There is no evidence to support a recommendation of necessity for supplements of protein to achieve the goals stated here. Food protein, particularly high-quality dairy protein, containing all EAA and high leucine may be more effective than other protein sources.
INTRODUCTION
The processes of muscle protein synthesis (MPS) and muscle protein breakdown (MPB) occur concurrently. This constant protein turnover allows the muscle fiber to change its protein structure if loading demands or diet changes. The plasticity of skeletal muscle to respond to altered loading and contractile patterns is evidence of the capacity for remodeling that a fiber can undergo. It is quite well documented for example that mitochondrial content increases with endurance-type work. In contrast, heavier loading leads to less change in mitochondrial content, but increases contractile or myofibrillar proteins. All of the aforementioned environmental adaptations represent a re-patterning of the muscle’s genetic expression patterns, protein translation, and processes for breakdown of existing protein structures to ‘insert’ the new proteins. A persistent muscle protein turnover also provides for a constant mechanism of protein ‘maintenance’ by removing damaged proteins and replacing them with new proteins. Damage to proteins can come about through oxidation or simply mechanical damage due to high forces during lengthening contractions. Regardless of the mechanism, the balance between the processes of muscle protein synthesis (MPS) and muscle protein breakdown (MPB) will determine the net gain, loss, or no change of proteins in the myofiber. On a gross level, protein balance determines whether muscle fiber size increases (hypertrophy) or is reduced (atrophy). Recent reviews highlight the regulation of the processes of protein synthesis (Philp et al., 2011) and protein breakdown (Glass, 2010), and the reader is referred to those publications for more precise information on the regulation of MPS and MPB, respectively.
What is well known is that in humans, performance of resistance exercise alone (Phillips et al., 1997) and consumption of protein or amino acids alone (Bohe et al., 2001) are both potent stimulators of MPS and mostly of the myofibrillar proteins (Moore et al., 2009b). A combination of resistance exercise and protein consumption, most usually as amino acids or protein consumed following resistance exercise, results in a synergistic stimulation of MPS (Biolo et al., 1997). Critically, the stimulation of MPS is thought to be a key process in the exercise-induced increment in muscle size that eventually sums to yield hypertrophy (Breen & Phillips, 2012; Churchward-Venne et al., 2012). Agreement between short-term changes in MPS or muscle net protein balance (i.e., MPS minus MPB) and long-term gains in muscle mass has been seen with nutrient provision (Hartman et al., 2007; Wilkinson et al., 2007) and differing contractile paradigms (Mitchell et al., 2012). In addition, it appears, at least in healthy humans and regardless of their age, that it is the meal-to-meal fluctuations in MPS that are far more influential in determining gains in (Breen & Phillips, 2012; Churchward-Venne et al., 2012) and losses of (Phillips et al., 2009) skeletal muscle mass. Thus, in a dynamic sense, undulations in MPS and only small changes in MPB determine net protein balance (Figure 1).

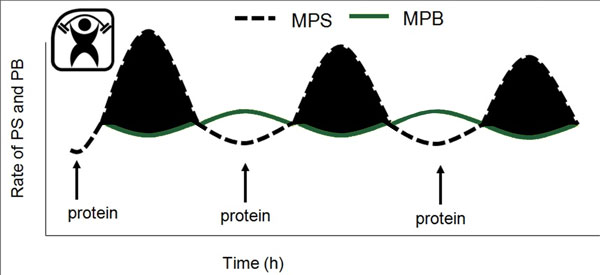
AMINO ACIDS: PROPERTIES AND DOSES IN THE STIMULATION OF MPS
Feeding protein or amino acids stimulates MPS, an effect that appears to be due almost exclusively to the amino acids themselves and not to changes in hormones such as insulin (Biolo et al., 1997; Fujita et al., 2007). It appears that only the essential amino acids (EAA) are required for this effect (Volpi et al., 2003). In particular, the amino acid leucine occupies a position of prominence since it alone acts as a stimulatory signal for MPS (Philp et al., 2011). It should be noted, however, that leucine cannot stimulate a rise in MPS in the absence of a full complement of EAA (Churchward-Venne et al., 2012). Thus, complete mixtures of amino acids (Tipton et al., 1999) or ingestion of intact proteins (Tipton et al., 2004; Wilkinson et al., 2007) are needed to demonstrate increases in MPS.
Interestingly, the process of MPS is saturable from a protein dose perspective. This would make sense since there is a finite capacity to store amino acids within muscle and no extra ‘reservoir’ of protein exists to accommodate excess protein nitrogen beyond that which can be used for amino-acid requiring processes. The dose-response of MPS appears to be a function of the extracellular amino acid concentrations rather than the intracellular (Bohe et al., 2003). The response of MPS in both young and older people was found to plateau at ~10 g of indispensable or essential amino acids (Cuthbertson et al., 2005). We also reported on the dose-response of MPS following resistance exercise using isolated egg protein as a dietary source (Moore et al., 2009a). What we observed, similar to what was reported previously (Cuthbertson et al., 2005), was that a plateau in MPS occurred at 20 g of ingested protein (~8.5 g of essential amino acids). Figure 2 shows a stylized plot of the response of MPS as well as a corresponding plot of leucine oxidation as an index of amino acid catabolism. What becomes evident is that as MPS plateaus, the ‘extra’ amino acids are simply being burned for fuel. Figure 2 also demonstrates that consuming very large quantities of amino acids and/or protein in the belief that this will accelerate traininginduced gains in muscle mass appears flawed. In contrast, ingesting a ‘mere’ 20 g of high-quality protein (0.25 g protein/kg body mass/ meal) will maximally stimulate MPS, the process that underpins changes in muscle mass. Of course, larger athletes (than the 87 kg subjects used in the Moore et al., 2009a study) may require more protein to maximize MPS and smaller athletes less.
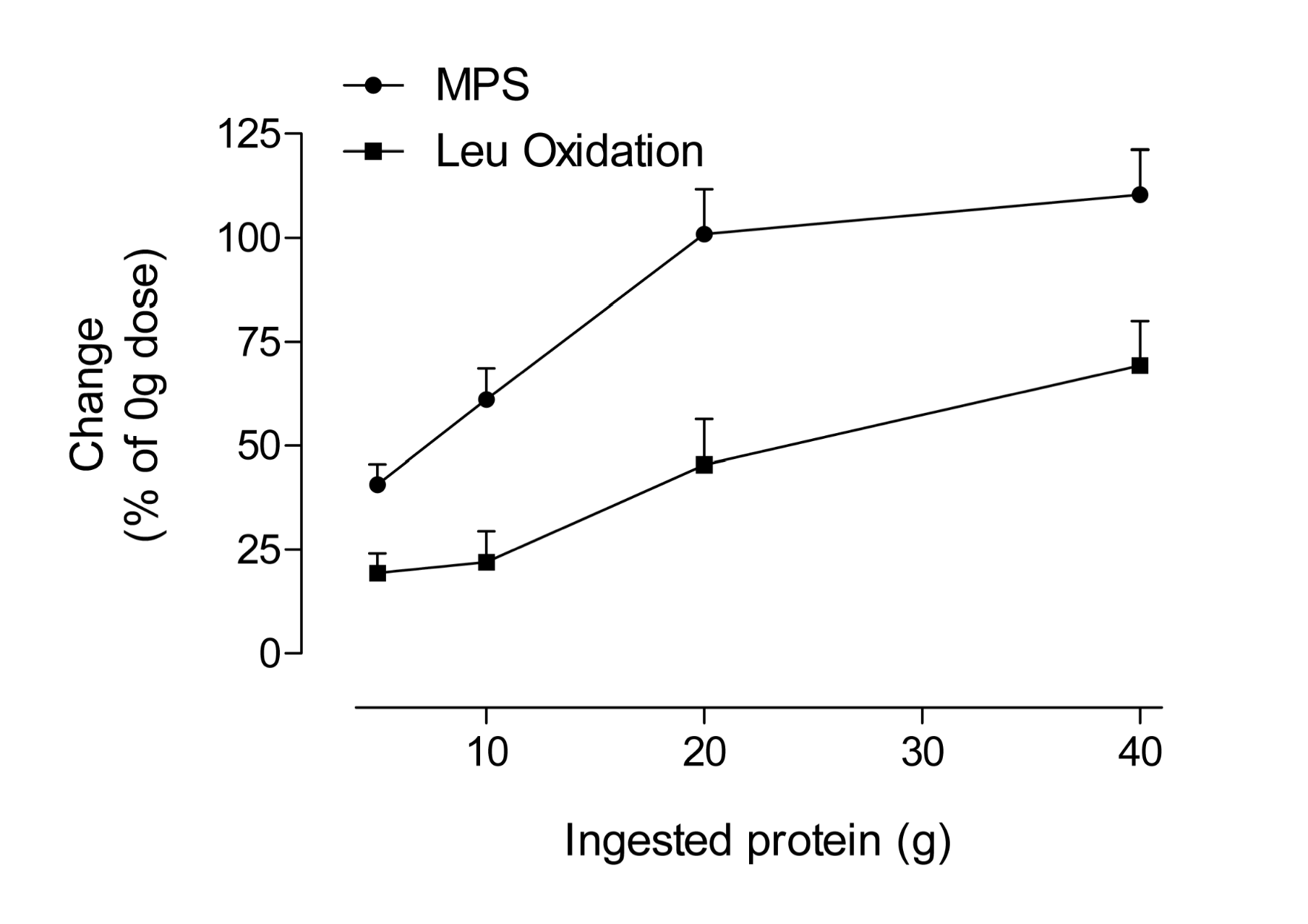
Figure 2: Percentage increases (from basal or 0 g) in muscle protein synthesis (MPS) and leucine oxidation after resistance exercise in young men as a function of ingested protein and leucine dose. The ingested protein was isolated egg protein; data extracted from (Moore et al., 2009a).
ACUTE NUTRITION AND EXERCISE INTERACTIONS
The effects of resistance exercise alone are potent and longlasting with basal fasted-state MPS being elevated for at least 48 h (Phillips et al., 1997). Feeding protein immediately post-exercise has been shown numerous times to stimulate MPS further (Breen & Phillips, 2012; Churchward-Venne et al., 2012). Even up to 24 h after resistance exercise muscle remains sensitive to the effects of resistance exercise and demonstrates an increased MPS response to increased blood amino acid levels (Burd et al., 2011). Thus, it is reasonable to propose that even at time points distal to 24 h, an enhanced sensitivity would be apparent (Figure 3).
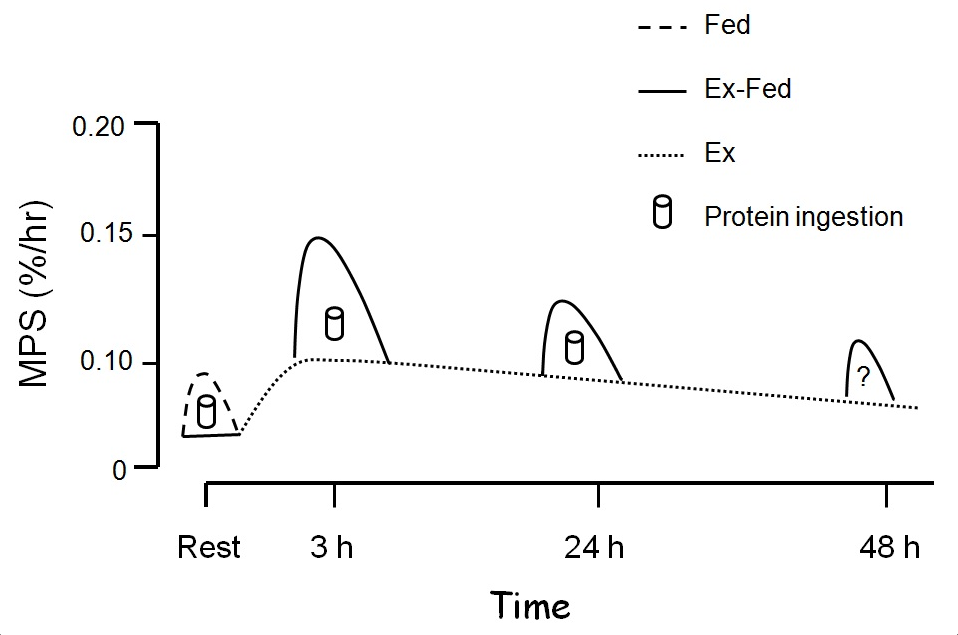
Figure 3: Resistance exercise stimulates a prolonged increase in muscle protein synthesis (MPS) that can remain elevated for ≥ 24 h (dashed lines). Thus, we propose that protein ingestion at any point during this enhanced period of ‘anabolic potential’ will be additive to these already elevated exercise mediated rates (solid line).
As Figure 3 illustrates the ‘window’ for protein consumption is longer than we perhaps appreciated. Nonetheless, there are likely advantages in consuming protein in close temporal proximity to exercise.
TIMING OF PROTEIN INGESTION
There is a physiological basis for why acute consumption of protein close to the performance of resistance exercise would have a stimulatory effect on muscle protein synthesis and lean mass building (Hartman et al., 2007; Holm et al., 2006). Increasing the amino acid concentration in the blood in conjunction with performance of resistance exercise would ‘take advantage’ of mechanisms at the cellular level that are activated following resistance exercise (Philp et al., 2011). Evidence exists to support the contention that consumption of protein (or amino acids), and not simply energy as carbohydrate, in close temporal proximity to resistance exercise is important to support greater hypertrophy (Hartman et al., 2007; Holm et al., 2006). These chronic training studies suggest the ‘window’ during which consumption of protein or amino acids should be consumed is likely <2 h after exercise in order to support greater increases in lean body mass and muscle hypertrophy in younger individuals. It is notable that in one acute study an equivalent full anabolic response in young individuals was mounted by skeletal muscle at both 1 h and 3 h post-exercise with crystalline amino acid consumption (Rasmussen et al., 2000). However, it has not been investigated whether this feeding pattern would translate into similar muscle hypertrophy with training. Therefore, in order to support greater hypertrophy with resistance training at any age it would be beneficial to consume a source of protein within 1 h after exercise cessation. In longer-term training type studies the effects of immediate (or at least temporally close) provision of nutrition appears to enhance muscle mass gains (Hartman et al., 2007; Holm et al., 2006). The impact of pre-exercise feeding received particular attention with the findings of Tipton et al. (2001) showing that pre-exercise consumption of amino acids, versus post-exercise, led to an enhanced post-exercise net amino acid balance. Importantly, however, two subsequent studies (Fujita et al., 2009; Tipton et al., 2006) could not reproduce the finding of a stimulatory effect of preexercise feeding (Tipton et al., 2001). Thus, it is equivocal at this time as to whether pre-exercise nutrition can effectively augment post-exercise anabolism.
PROTEIN SOURCE: IMPACT ON MPS
Acute studies in which subjects have consumed whole milk proteins or soy protein (Elliot et al., 2006; Wilkinson et al., 2007), both in isolation or as a supplement (Tipton et al., 2004; Tipton et al., 2006), have all shown that these proteins are able to support muscle protein accretion following resistance exercise. For example, the ability of fat-free fluid milk (237 mL, 377 kJ, 8.8 g protein), whole milk (237 mL, 627 kJ, 8.0 g protein), and an isoenergetic amount (to the whole milk) of fat-free milk (393 mL, 626 kJ, 14.5 g protein) to support post-exercise muscle building were compared (Elliot et al., 2006). It was found that whole milk resulted in greater threonine, and a trend for greater phenylalanine, net uptake across an exercised leg, suggesting whole milk was superior to fat-free fluid milk in its ability to build muscle after exercise. The long-term consequences of consuming isonitrogenous quantities of fat-free versus whole milk would suggest that whole milk should result in greater protein accretion, but we lack the long-term studies to make that conclusion at present. When the consumption of fat-free fluid milk (500 mL, 745 kJ, 18.2 g protein) vs. an isonitrogenous, isoenergetic, and macronutrient composition-matched amount of a soy protein beverage was compared, a greater net muscle protein balance and fractional synthetic rate with milk ingestion was reported (Wilkinson et al., 2007). It was hypothesized that these findings resulted from differences in protein digestion rate which affected the amino acid increase in the blood and subsequently impacted muscle protein anabolism. This is supported by data from studies documenting differences in how milk and soy proteins are partitioned for use between the splanchnic and peripheral (i.e., muscle) tissues (Fouillet et al., 2002). Specifically, soy proteins support greater splanchnic protein synthesis and are converted to urea to a greater extent than are milk proteins (Fouillet et al., 2002). A rapidly digested whey protein hydrolysate was more effective than both soy and micellar casein (the form of casein in milk) in stimulating MPS both at rest and following resistance exercise and the response of MPS correlated with the peak leucine concentration (Tang et al., 2009). This observation was something recently corroborated (Pennings et al., 2011) with virtually identical findings with whey ingestion. These data (Pennings et al., 2011; Tang et al., 2009) lead to the proposal of the ‘leucine trigger’ hypothesis (Figure 4), which simply stated would predict that the more rapidly digested protein that contained a high proportion of leucine would be most effective at promoting increases in MPS (Breen & Phillips, 2012; Churchward-Venne et al., 2012).
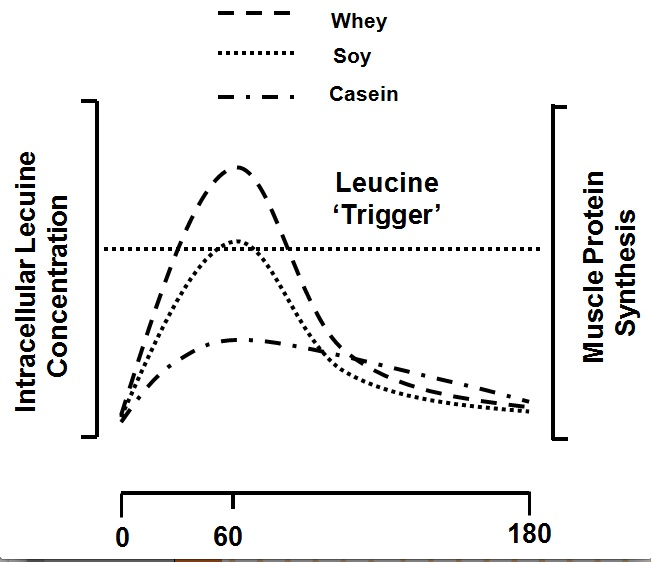
Figure 4: The ‘leucine trigger’ concept with data adapted from (Tang et al., 2009) as shown for whey protein, soy protein, and casein proteins. These proteins would be digested in the following order: whey ≥ soy >> casein, and the following leucine content: whey > casein > soy. Thus, a greater and more rapid rise in blood leucine triggers a greater rise in MPS.
In addition to both hydration and carbohydrate the importance of protein needs to be recognized for its role in allowing adaptation and recovery. Excessively large protein intakes do not appear to assist in promoting adaptation and may compromise the intake of other important nutrients such as carbohydrate. At the levels recommended here, and with particular attention to protein consumption in the post-exercise time period, athletes should be able to maximize their muscle recovery, and likely that of the connective tissues, and in doing so allow performance to their peak potential.
PRACTICAL APPLICATIONS
- Consume protein after exercise to maximize protein synthesis and promote adaptation.
- Liquid forms of protein are best due to their rapid digestion rate.
- Rapidly digested proteins are best with isolated proteins such as whey, milk proteins, or soy appearing to be most efficient.
- Whey protein, due to its leucine content, represents, on a per g basis, the best protein source to stimulate new muscle protein synthesis.
- Consume protein throughout the day at regularly spaced intervals to maximize the anabolic response (20-25 g per meal or between 0.25-0.30 g protein/kg body mass/meal).
- Excessive quantities of protein are not necessary for performance, however, levels of protein intake in the range of 1.2-1.6 g protein/ kg/d would be adequate and more than required by most athletes even during heavy training.
REFERENCES
Biolo, G., K. D. Tipton, S. Klein, and R. R. Wolfe (1997). An abundant supply of amino acids enhances the metabolic effect of exercise on muscle protein. Am. J. Physiol. 273: E122-E129.
Bohe, J., J. F. Low, R. R. Wolfe, and M. J. Rennie (2001). Latency and duration of stimulation of human muscle protein synthesis during continuous infusion of amino acids. J. Physiol. 532: 575-579.
Bohe, J., A. Low, R. R. Wolfe, and M. J. Rennie (2003). Human muscle protein synthesis is modulated by extracellular, not intramuscular amino acid availability: a dose-response study. J. Physiol. 552: 315-324.
Breen, L., and S. M. Phillips (2012). Nutrient interaction for optimal protein anabolism in resistance exercise. Curr. Opin. Clin. Nutr. Metab. Care 15: 226-232.
Burd, N.A., D. W. West, D. R. Moore, P. J. Atherton, A. W. Staples, T. Prior, J. E. Tang, M. J. Rennie, S. K. Baker, and S. M. Phillips (2011). Enhanced amino acid sensitivity of myofibrillar protein synthesis persists for up to 24 h after resistance exercise in young men. J. Nutr. 141: 568-573.
Churchward-Venne, T.A., N. A. Burd, and S. M. Phillips (2012). Nutritional regulation of muscle protein synthesis with resistance exercise: strategies to enhance anabolism. Nutr. Metab. (Lond.) 9: 40.
Cuthbertson, D., K. Smith, J. Babraj, G. Leese, T. Waddell, P. Atherton, H. Wackerhage, P. M. Taylor, and M. J. Rennie (2005). Anabolic signaling deficits underlie amino acid resistance of wasting, aging muscle. FASEB J. 19: 422-424.
Elliot, T.A., M. G. Cree, A. P. Sanford, R. R. Wolfe, and K. D. Tipton (2006). Milk ingestion stimulates net muscle protein synthesis following resistance exercise. Med. Sci. Sports Exerc. 38: 667-674.
Fouillet, H., F. Mariotti, C. Gaudichon, C. Bos, and D. Tome (2002). Peripheral and splanchnic metabolism of dietary nitrogen are differently affected by the protein source in humans as assessed by compartmental modeling. J. Nutr. 132: 125-133.
Fujita, S., H. C. Dreyer, M. J. Drummond, E. L. Glynn, J. G. Cadenas, F. Yoshizawa, E. Volpi, and B. B. Rasmussen (2007). Nutrient signalling in the regulation of human muscle protein synthesis. J. Physiol. 582: 813-823.
Fujita, S., H. C. Dreyer, M. J. Drummond, E. L. Glynn, E. Volpi, and B. B. Rasmussen (2009). Essential amino acid and carbohydrate ingestion before resistance exercise does not enhance postexercise muscle protein synthesis. J. Appl. Physiol. 106: 1730-1739.
Glass, D.J. (2010). Signaling pathways perturbing muscle mass. Curr. Opin. Clin. Nutr. Metab. Care 13: 225-229.
Hartman, J.W., J. E. Tang, S. B. Wilkinson, M. A. Tarnopolsky, R. L. Lawrence, A. V. Fullerton, and S. M. Phillips (2007). Consumption of fat-free fluid milk after resistance exercise promotes greater lean mass accretion than does consumption of soy or carbohydrate in young, novice, male weightlifters. Am. J. Clin. Nutr. 86: 373-381.
Holm, L., B. Esmarck, M. Mizuno, H. Hansen, C. Suetta, P. Holmich, M. Krogsgaard, and M. Kjaer (2006). The effect of protein and carbohydrate supplementation on strength training outcome of rehabilitation in ACL patients. J. Orthop. Res. 24:2114-2123.
Karlsson, H.K., P. A. Nilsson, J. Nilsson, A. V. Chibalin, J. R. Zierath, and E. Blomstrand (2004). Branched-chain amino acids increase p70S6k phosphorylation in human skeletal muscle after resistance exercise. Am. J. Physiol. 287: E1-E7.
Mitchell, C.J., T. A. Churchward-Venne, D. D. West, N. A. Burd, L. Breen, S. K. Baker, and S. M. Phillips (2012). Resistance exercise load does not determine training-mediated hypertrophic gains in young men. J Appl. Physiol. 113:71-77.
Moore D.R., M. J. Robinson, J. L. Fry, J. E. Tang, E. I. Glover, S. B. Wilkinson, T. Prior, M. A. Tarnopolsky, and S. M. Phillips (2009a). Ingested protein dose response of muscle and albumin protein synthesis after resistance exercise in young men. Am. J. Clin. Nutr. 89: 161-168.
Moore, D.R., J. E. Tang, N. A. Burd, T. Rerecich, M. A. Tarnopolsky, and S. M. Phillips (2009b). Differential stimulation of myofibrillar and sarcoplasmic protein synthesis with protein ingestion at rest and after resistance exercise. J. Physiol. 597: 897-904.
Pennings, B., Y. Boirie, J. M. Senden, A. P. Gijsen, H. Kuipers, and L. J. van Loon (2011). Whey protein stimulates postprandial muscle protein accretion more effectively than do casein and casein hydrolysate in older men. Am. J. Clin. Nutr. 93: 997-1005.
Phillips, S.M., K. D. Tipton, A. Aarsland, S. E. Wolf, and R. R. Wolfe (1997). Mixed muscle protein synthesis and breakdown after resistance exercise in humans. Am. J. Physiol. 273: E99-E107.
Phillips, S.M., E. I. Glover, and M. J. Rennie (2009). Alterations of protein turnover underlying disuse atrophy in human skeletal muscle. J. Appl. Physiol. 107: 643-654.
Philp A., D. L. Hamilton, and K. Baar (2011). Signals mediating skeletal muscle remodeling by resistance exercise: PI3-kinase independent activation of mTORC1. J. Appl. Physiol. 110: 561-568.
Rasmussen, B.B., K. D. Tipton, S. L. Miller, S. E. Wolf, and R. R. Wolfe (2000). An oral essential amino acid-carbohydrate supplement enhances muscle protein anabolism after resistance exercise. J. Appl. Physiol. 88: 386-392.
Tang, J.E., D. R. Moore, G. W. Kujbida, M. A. Tarnopolsky, and S. M. Phillips (2009). Ingestion of whey hydrolysate, casein, or soy protein isolate: effects on mixed muscle protein synthesis at rest and following resistance exercise in young men. J. Appl. Physiol. 107: 987-992.
Tipton, K.D., A. A. Ferrando, S. M. Phillips, D. Doyle, Jr., and R. R. Wolfe (1999). Postexercise net protein synthesis in human muscle from orally administered amino acids. Am. J. Physiol. 276: E628-E634.
Tipton, K.D., B. B. Rasmussen, S. L. Miller, S. E. Wolf, S. K. Owens-Stovall, B. E. Petrini, and R. R. Wolfe (2001). Timing of amino acid-carbohydrate ingestion alters anabolic response of muscle to resistance exercise. Am. J. Physiol. 281: E197-E206. Tipton, K.D., T. A. Elliott, M. G. Cree, S. E. Wolf, A. P. Sanford, and R. R. Wolfe (2004). Ingestion of casein and whey proteins result in muscle anabolism after resistance exercise. Med. Sci. Sports Exerc. 36: 2073-2081.
Tipton, K.D., T. A. Elliott, M. G. Cree, A. A. Aarsland, A. P. Sanford, and R. R. Wolfe (2006). Stimulation of Net Muscle Protein Synthesis by Whey Protein Ingestion Before and After Exercise. Am. J. Physiol. 292: E71-E76.
Volpi, E., H. Kobayashi, M. Sheffield-Moore, B. Mittendorfer, and R. R. Wolfe (2003). Essential amino acids are primarily responsible for the amino acid stimulation of muscle protein anabolism in healthy elderly adults. Am. J. Clin. Nutr. 78: 250-258.
Wilkinson, S.B., M. A. Tarnopolsky, M. J. MacDonald, J. R. Macdonald, D. Armstrong, and S. M. Phillips (2007). Consumption of fluid skim milk promotes greater muscle protein accretion following resistance exercise than an isonitrogenous and isoenergetic soy protein beverage. Am. J. Clin. Nutr. 85: 1031-1040.