KEY POINTS
- Very few sports use only endurance or strength. Outside of running long distances on a flat surface and powerlifting, practically all sports require some combination of endurance and strength.
- Endurance and strength can be developed simultaneously to some degree. However, as the frequency and intensity of endurance training increases, the development or maintenance of muscle mass and strength is slowed. This interaction between endurance and strength is called the concurrent training effect.
- Increasing strength and muscle mass requires the activation of the mammalian target of rapamycin (mTOR). The signaling molecule mTOR is activated maximally by lifting heavy weights to failure and consuming leucine-rich proteins.
- Endurance adaptations occur when metabolic stress is highest (energy supply is low and energy demand is high). Metabolic stress activates the adenosine monophosphate-activated protein kinase (AMPK) and the nicotinamide adenine dinucleotide (NAD)-dependent deacetylase sirtuin 1 (SIRT1).
- The concurrent training effect can be explained at the molecular level, in part by the fact that metabolic stress (increased AMPK and SIRT1 activities) can inhibit the activation of mTOR and muscle hypertrophy.
- By understanding: a) the importance of mTOR in the development of muscle mass and strength; b) the time course of mTOR activation; and c) the role of nutrition in mTOR/AMPK/SIRT1 activation, a simple training and nutritional plan can be developed to maximize strength and endurance.
INTRODUCTION TO THE SCIENCE OF CONCURRENT TRAINING
Robert Hickson was a powerlifter when he went to do his postdoctoral work in the laboratory of Professor John Holloszy, the “father of endurance exercise research.” To make a good impression, Dr. Hickson accompanied his new boss on his afternoon runs, but soon found that his muscle mass and strength were decreasing in spite of strength training at the same frequency and intensity. When Hickson approached Holloszy with his problem, he was told, “This should be the first study you do when you have your own laboratory.” True to his word, the first study that Hickson completed in his new laboratory at the University of Illinois in Chicago was the seminal study on concurrent training.
Published in 1980, Hickson’s classic study trained three groups of subjects: Group 1 performed strength training alone; Group 2 performed endurance training alone; and Group 3 performed strength and endurance training together. The strength training was performed 5 d/wk for 10 wk, and was designed exclusively to increase leg strength. True to his powerlifting background, Hickson had his subjects perform all of the exercises with as much weight as possible. The endurance training was performed 6 d/wk for the same 10-wk period and consisted of 3 d of cycling and 3 d of running. The cycling exercise consisted of six 5-min intervals at maximal oxygen uptake (VO2max), whereas the instructions on the running days were to “run as fast as possible” for 30 min/day in the first week, 35 min/day for the second week and 40 min/day for the remainder of the study. The concurrent training group performed both the strength and endurance training protocols in a non-standardized order with between 15 min and 2 h of rest in between.
At the end of the 10-wk training program, VO2max was determined on the bike and treadmill. The strength alone group showed a 4% improvement in VO2max on the bike with no change in max when measured on the treadmill. In contrast, the endurance and concurrent training groups both increased VO2max 17% on the treadmill and ~20% on the bike. This indicated that strength training did not negatively affect endurance adaptations or performance. It should be noted however, that the concurrent training group did not increase their bodyweight over the training period as a result of their strength training. If they had, we would expect that the energetic cost of exercise would rise (Roberts et al., 1998). The rise in the energetic cost of covering a given distance (decreased economy) would decrease endurance performance, especially during running where they would have to support and propel this extra mass.
Average strength in the strength training only and concurrent training groups increased at the same rate throughout the first 6-7 wk of training (Figure 1). Strength continued to increase throughout the entire 10-wk training period in the strength training only group. In contrast, strength leveled off between the 7th and 8th wk in the concurrent training group and surprisingly decreased during the 9th and 10th wk of
training. This indicated that high-intensity endurance exercise of a sufficient frequency can inhibit long-term strength adaptations.
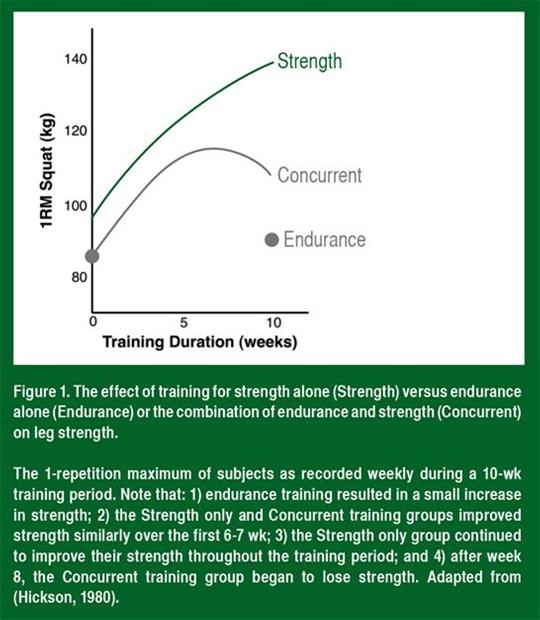
When others repeated the frequency and intensity that Hickson employed in his study, they reported similar decreases in strength gains and impaired muscle fiber hypertrophy (Kraemer et al., 1995; Wilson et al., 2012). For example, Kraemer and colleagues (1995) showed that running and strength training at a high intensity for 4 d/wk resulted in smaller gains in power and impaired muscle fiber hypertrophy compared to training for strength alone. Strength training alone resulted in ~28% hypertrophy, whereas concurrent training resulted in hypertrophy of only ~16%. This indicated that concurrent endurance training impaired not only strength, but muscle hypertrophy as well.
Not every study on concurrent exercise shows that endurance blocks strength adaptations. In fact, studies where the frequency or the intensity of training is decreased did not find any interference effect. For example, in two separate studies McCarthy and colleagues demonstrated that cycling 3 d/wk for 50 min at 70% VO2max was not enough to impair strength (1995) or hypertrophy (2002) as a result of concurrent strength training. These data suggested that strength and endurance could increase together up to a point. However, once the frequency increases past 4 d/wk or the intensity of endurance exercise increases above 80% VO2max, endurance exercise slows or limits the increase in muscle mass and strength that occurs with strength training. This was illustrated nicely in a recent meta-analysis that demonstrated that the effect sizes of strength training alone on muscle hypertrophy and strength were 1.22 and 1.71, respectively (Wilson et al., 2012). The corresponding numbers for concurrent training were 0.8 and 1.28, indicating that, in a large cohort, endurance exercise impairs muscle size and strength adaptations.
MOLECULAR UNDERPINNING OF THE CONCURRENT TRAINING EFFECT
Increased strength is the combined effect of improvements in neural activation, muscle fiber size and connective tissue stiffness. Therefore, concurrent endurance exercise could decrease adaptations of any or all of these physiological parameters. There does not appear to be a decrease in the neural (learning) adaptation since in the early stages of training, when the neural adaptation is the strongest (4, 6 and 8 wk; see Figure 1), strength is similar between strength and concurrent training groups (Hickson, 1980; Kraemer et al., 1995). To date, no one has measured the effect of concurrent training on connective tissue stiffness, so we are unsure of the role of this tissue in the impaired strength response. However, as stated above, there is evidence that muscle hypertrophy is impaired in individuals training at a high intensity for both strength and endurance together, compared with those training exclusively with strength exercises, and this correlated quite well with the impaired strength response (Kraemer et al., 1995; Wilson et al., 2012). Therefore, the primary effect of endurance exercise seems to be a decrease in resistance exercise-induced muscle hypertrophy.
Previous Sports Science Exchange articles (Baar, 2013; Baar, 2014) have discussed the molecular events that lead to muscle hypertrophy and increased endurance capacity. In brief, these studies have shown that for exercise-induced muscle hypertrophy, the key signaling molecule is the mammalian target of rapamycin (mTOR), whereas endurance adaptations result from metabolic stress as sensed by proteins such as the calcium-calmodulin kinases (CaMK), AMP-activated protein kinase (AMPK), the 38KDa mitogen-activated protein kinase (p38) and the nicotinamide adenine dinucleotide (NAD+)-dependent deacetylase family of sirtuins (SIRT).
mTOR is a serine/threonine protein kinase that regulates the rate of protein synthesis. In all non-diseased people and animals studied to date (Figure 2), mTOR activity (as determined by the phosphorylation of S6K1) correlates with muscle hypertrophy (Baar & Esser, 1999; Terzis et al., 2008). Furthermore, the mTOR-specific inhibitor rapamycin blocks both the acute increase in protein synthesis after strength training (Drummond et al., 2009) and muscle hypertrophy following loading (Goodman et al., 2011). These data suggest that the activation of mTOR is required for muscle hypertrophy following strength training.
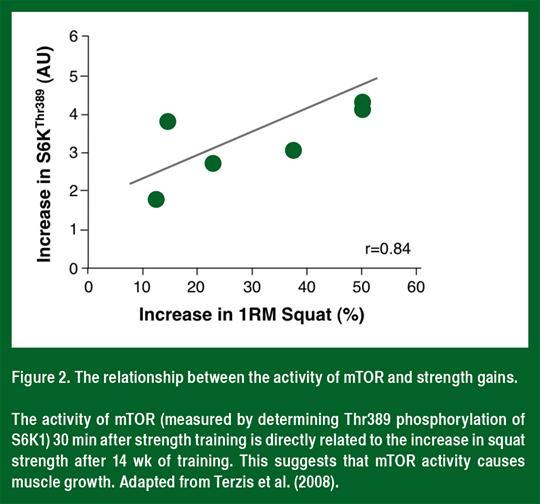
High-intensity endurance exercise results in the activation of CaMK, AMPK, p38 and SIRT1. All of these proteins increase the amount and/or the activity of the peroxisome proliferator g coactivator 1a (PGC-1a), which is a transcriptional co-factor that increases mitochondrial mass and the number of capillaries in muscle. Even though all of these proteins are activated by exercise, the activation of AMPK (by the rise in free AMP) and SIRT1 (by the increase in NAD flux; i.e., lactate production) are most closely associated with high-intensity training and therefore the most likely candidates to block muscle hypertrophy.
The first hint of a molecular mechanism that could explain how endurance exercise impaired muscle hypertrophy of concurrent strength training came when metabolic stress was found to block mTOR activity (Inoki et al., 2003). Over the last few years, it has become clear that metabolic stress can block mTOR through: 1) AMPK phosphorylating and activating the mTOR inhibitor tublero sclerosis complex (TSC2) (Inoki et al., 2003); 2) AMPK phosphorylating and inhibiting the mTOR regulator raptor (Gwinn et al., 2008); and 3) AMPK-independent prevention of mTOR localization to the lysosome (Kim et al., 2013).
Putting together the effect of metabolic stress/AMPK activation on mTOR and the fact that metabolic stress and AMPK activity were increased during endurance exercise, exercise physiologists began to ask the question: “Can AMPK limit muscle hypertrophy?” Thomson and Gordon (2005) were the first to show that impaired muscle growth occurred in rats where AMPK activity was elevated, supporting the hypothesis that AMPK mediated the concurrent training effect. They went further, using a drug to activate AMPK in muscles before resistance exercise, and consistent with the hypothesis, blocked mTOR activation (Thomson et al., 2008). Therefore, high AMPK activity can inhibit mTOR activation in animals.
John Hawley’s laboratory (Figure 3) has shown that mTOR activity is inhibited in humans following ten, 6 s maximal sprints, but not following 30 min of moderate intensity cycling (Coffey et al., 2009a, b).
Consistent with endurance exercise intensity being a key to the interference effect, Lundberg et al. (2012) did not find any inhibition of mTOR activation when subjects performed only 45 min of cycling at 70% VO2max, 6 h before performing resistance exercise. Further, Apró and his colleagues (2013) did not report a decrease in mTOR signaling when subjects performed 30 min of cycling at 70% of VO2max, 15 min after completing a resistance training session. These findings are completely consistent with the training data that shows that the interference effect is only present if the subjects train at a high frequency and intensity (Hickson, 1980; Kraemer et al., 1995).
Even though the intensity effects and the animal data are completely consistent with AMPK mediating the inhibition of mTOR activity during concurrent training, in the sprint interval study by Coffey and colleagues (2009a), the activation of AMPK in both of the training groups was the same, suggesting that AMPK could not explain the inhibition of mTOR activity. This indicated that, even though high-intensity endurance exercise can inhibit mTOR and muscle hypertrophy, other proteins (possibly SIRT1) contribute to the molecular mechanisms underlying the concurrent training effect.
SCIENCE-BASED RECOMMENDATIONS FOR TRAINING TO MAXIMIZE CONCURRENT TRAINING
Using the molecular information provided above, some simple nutritional and training strategies can be devised to maximize the adaptations to concurrent training. The goal of these recommendations is to maximize the mitochondrial adaptation to endurance exercise and the muscle mass and strength adaptations to strength training. To do this, it is recommended that:
- High-intensity endurance training sessions should be performed early in the day. A period of recovery of at least 3 h should be given so that metabolic stress can return to baseline levels before resistance exercise is performed. This suggestion is based on the fact that AMPK activity increases rapidly and then returns to baseline levels within the first 3 h after high-intensity exercise (Wojtaszewski et al., 2000), whereas mTOR activity can be maintained for at least 18 h after resistance exercise (Baar & Esser, 1999).
- Fully refuel with carbohydrate between the morning high-intensity endurance training session and the afternoon strength session since AMPK can be activated by low glycogen stores (McBride et al., 2009), and SIRT1 is activated by caloric restriction (Schenk et al., 2011).
- If it is not possible to refuel completely because in-season training volume and intensity is too high, it might be better to reserve a portion of the offseason (and short in-season periods) exclusively for increasing muscle size and strength and then use higher dietary protein intakes to maintain that muscle mass as the aerobic load increases through the season (Mettler et al., 2010).
- Resistance exercise should be supported by 0.25 g/kg of readily digestible, leucine-rich protein as soon as possible after training and every four hours thereafter. Since, in this scenario, resistance exercise is performed later in the day, it becomes even more important to also consume protein immediately prior to sleep to maximize the synthetic response overnight (Res et al., 2012).
- To improve the endurance response to lower-intensity endurance training sessions and provide a strong strength stimulus, consider performing strength training immediately after low-intensity, non-glycogen depleting endurance sessions. Performing a strength session immediately after a low-intensity endurance session results in a greater stimulus for endurance adaptation than the low-intensity endurance session alone (Wang et al., 2011) and the low-intensity session will not block mTOR (Apró et al., 2013; Coffey et al., 2009b; Lundberg et al., 2012).
CONCLUSIONS
These simple recommendations, based on our current understanding of the molecular response to exercise, should allow for the maximal adaptive response to both endurance and strength exercise. However, improving endurance and strength together in an elite athlete is more than just striking the right balance between AMPK and mTOR. This is especially true in situations where performance is based on skill optimization that goes well beyond these simple molecular pathways. In the end, how an athlete performs with their improved endurance and strength is based on far more complex processes, which are unfortunately poorly understood.
REFERENCES
Apró, W., L. Wang, M. Ponten, E. Blomstrand, and K. Sahlin (2013). Resistance exercise induced mTORC1 signaling is not impaired by subsequent endurance exercise in human skeletal muscle. Am. J. Physiol. 305:E22-E32.
Baar, K (2013). New ideas about nutrition and the adaptation to endurance training. Sports Science Exchange. 115:1-5.
Baar, K (2014). Nutrition and the molecular response to strength training. Sports Science Exchange. 123:1-4.
Baar, K., and K. Esser (1999). Phosphorylation of p70(S6K) correlates with increased skeletal muscle mass following resistance exercise. Am. J. Physiol. 276:C120-C127.
Coffey, V.G., B. Jemiolo, J. Edge, A.P. Garnham, S.W. Trappe, and J.A. Hawley. (2009a). Effect of consecutive repeated sprint and resistance exercise bouts on acute adaptive responses in human skeletal muscle. Am. J. Physiol. 297:R1441-R1451.
Coffey, V.G., H. Pilegaard, A.P. Garnham, B.J. O'Brien, and J.A. Hawley (2009b). Consecutive bouts of diverse contractile activity alter acute responses in human skeletal muscle. J. Appl. Physiol. 106:1187-1197.
Drummond, M.J., C.S. Fry, E.L. Glynn, H.C. Dreyer, S. Dhanani, K.L. Timmerman, E. Volpi, and B.B. Rasmussen (2009). Rapamycin administration in humans blocks the contraction-induced increase in skeletal muscle protein synthesis. J. Physiol. 587:1535-1546.
Goodman, C.A., J. W. Frey, D.M. Mabrey, B.L. Jacobs, H.C. Lincoln, J.S. You, and T.A. Hornberger (2011). The role of skeletal muscle mTOR in the regulation of mechanical load-induced growth. J. Physiol. 589:5485-5501.
Gwinn, D.M., D.B. Shackelford, D.F. Egan, M.M. Mihaylova, A. Mery, D.S. Vasquez, B.E. Turk, and R.J. Shaw (2008). AMPK phosphorylation of raptor mediates a metabolic checkpoint. Mol. Cell. 30:214-226.
Hickson, R.C. (1980). Interference of strength development by simultaneously training for strength and endurance. Eur. J. Appl. Physiol. Occup. Physiol. 45:255-263.
Inoki, K., T. Zhu, K.L. Guan, (2003). TSC2 mediates cellular energy response to control cell growth and survival. Cell 115:577-590.
Kim, S.G., G.R. Hoffman, G. Poulogiannis, G.R. Buel, Y.J. Jang, K.W. Lee, B.Y. Kim, R.L. Erikson, L.C. Cantley, A.Y. Choo, and J. Blenis (2013). Metabolic stress controls mTORC1 lysosomal localization and dimerization by regulating the TTT-RUVBL1/2 complex. Mol. Cell.49:172-185.
Kraemer, W.J., J.F. Patton, S.E. Gordon, E.A. Harman, M.R. Deschenes, K. Reynolds, R.U. Newton, N.T. Triplett NT, and J.E. Dziados. (1995). Compatibility of high-intensity strength and endurance training on hormonal and skeletal muscle adaptations. J. Appl. Physiol. 78:976-989.
Lundberg, T.R., R. Fernandez-Gonzalo, T. Gustafsson, and P.A. Tesch (2012). Aerobic exercise alters skeletal muscle molecular responses to resistance exercise. Med. Sci. Sports Exerc.44:1680-1688.
McBride, A., S. Ghilagaber, A. Nikolaev,and D.G. Hardie (2009). The glycogen-binding domain on the AMPK beta subunit allows the kinase to act as a glycogen sensor. Cell Metab. 9:23-34.
McCarthy, J.P., J.C. Agre, B.K. Graf, M.A. Pozniak, and A.C. Vailas (1995). Compatibility of adaptive responses with combining strength and endurance training. Med Sci. Sports Exerc.27:429-436.
McCarthy, J.P., M.A. Pozniak, and J.C. Agre (2002). Neuromuscular adaptations to concurrent strength and endurance training. Med. Sci. Sports Exerc. 34:511-519.
Mettler, S., N. Mitchell, and K.D. Tipton (2010). Increased protein intake reduces lean body mass loss during weight loss in athletes. Med. Sci. Sports Exerc. 42:326-337.
Res, P.T., B. Groen, B. Pennings, M. Beelen, G.A. Wallis, A. P. Gijsen, J.M. Senden, and L.J. van Loon. (2012). Protein Ingestion Prior To Sleep Improves Post-Exercise Overnight Recovery.Med. Sci. Sports Exerc. 44:1560-1569.
Roberts, T.J., R. Kram, P.G. Weyand, and C.R. Taylor (1998). Energetics of bipedal running. I. Metabolic cost of generating force. J. Exp. Biol. 201:2745-2751.
Schenk, S., C.E. McCurdy, A. M.Z. Phi Chen, M.J. Holliday, G.K. Bandyopadhyay, O. Osborn, K. Baar, and J.M. Olefsky (2011). Sirt1 enhances skeletal muscle insulin sensitivity in mice during caloric restriction. J. Clin. Invest. 121:4281-4288.
Terzis, G., G. Georgiadis, G. Stratakos, I. Vogiatzis, S. Kavouras, P. Manta, H. Mascher, and E. Blomstrand (2008). Resistance exercise-induced increase in muscle mass correlates with p70S6 kinase phosphorylation in human subjects. Eur. J. Appl. Physiol. 102:145-152.
Thomson, D.M., and S.E. Gordon (2005). Diminished overload-induced hypertrophy in aged fast-twitch skeletal muscle is associated with AMPK hyperphosphorylation. J. Appl. Physiol.98:557-564.
Thomson, D.M., C.A. Fick, and S.E. Gordon (2008). AMPK activation attenuates S6K1, 4E-BP1, and eEF2 signaling responses to high-frequency electrically stimulated skeletal muscle contractions. J. Appl. Physiol. 104:625-632.
Wang, L., H. Mascher, N. Psilander, E. Blomstrand, and K. Sahlin (2011). Resistance exercise enhances the molecular signaling of mitochondrial biogenesis induced by endurance exercise in human skeletal muscle. J. Appl. Physiol. 111:1335-1344.
Wilson, J.M., P.J. Marin, M.R. Rhea, S.M. Wilson J.P. Loenneke, and J.C. Anderson (2012). Concurrent training: a meta-analysis examining interference of aerobic and resistance exercises. J. Strength Cond. Res. 26:2293-2307.
Wojtaszewski, J.F., P. Nielsen, B.F. Hansen, E.A. Richter, and B Kiens (2000) Isoform-specific and exercise intensity-dependent activation of 5'-AMP-activated protein kinase in human skeletal muscle. J. Physiol. 528:221-226.